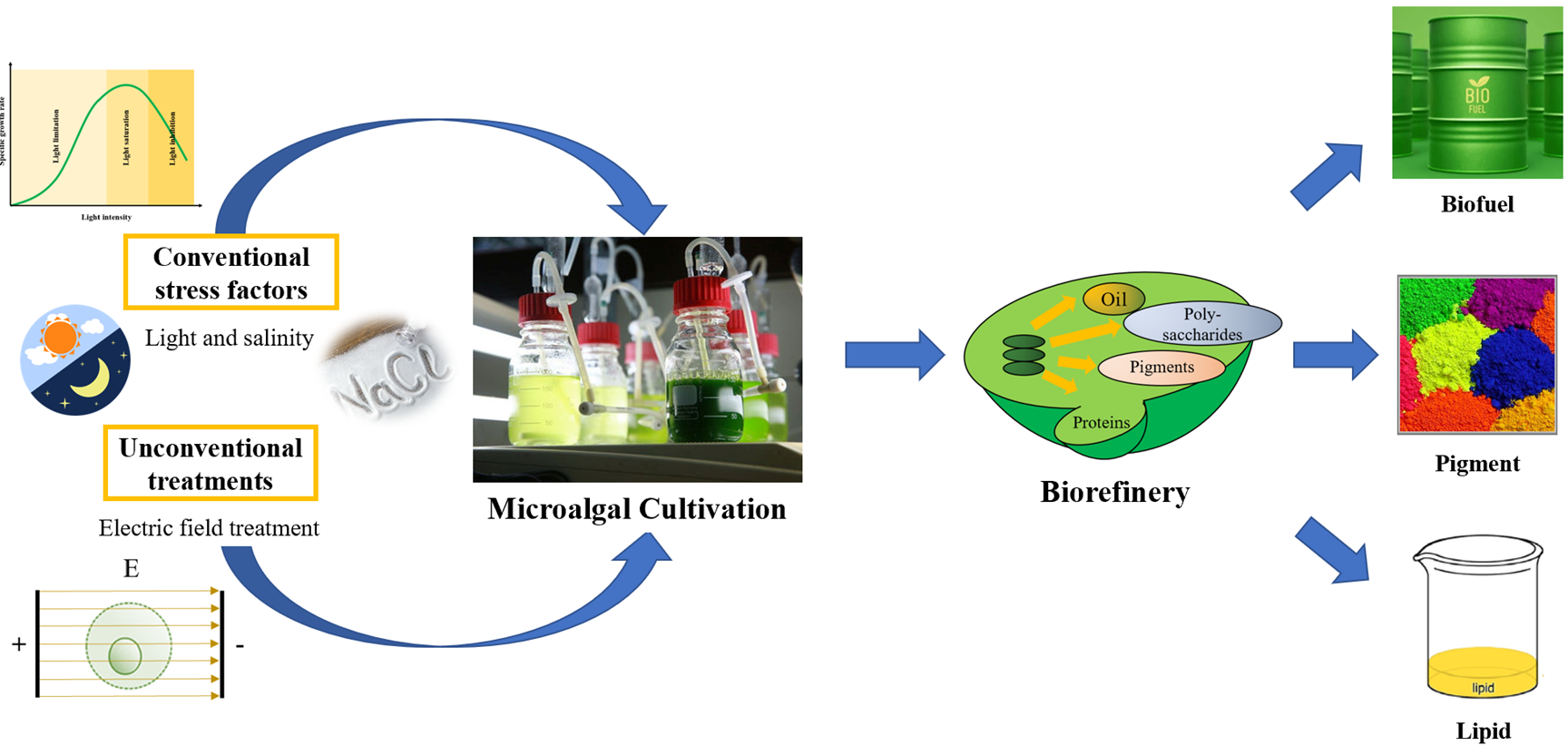
Downloads
Download


This work is licensed under a Creative Commons Attribution 4.0 International License.
Review
Harnessing Stress: Conventional and Unconventional Strategies for Enhancing Microalgal Productivity in Sustainable Biorefineries
Chia-En Chuang 1,†, Yu-Han Chien 1,†, Sheng-Yung Lin 1,†, Adi Kusmayadi 2, Jia-Hui Zhou 1,
Chiao Ching Chang 1, Chen Rui Zhang 1 and Yoong Kit Leong 1,3,*
1 Department of Chemical and Materials Engineering, Tunghai University, Taichung 407, Taiwan
2 Department of Mechanical Engineering, Politeknik Negeri Indramayu, Indramayu 45252, Indonesia
3 Research Center for Smart Sustainable Circular Economy, Tunghai University, Taichung 407, Taiwan
* Correspondence: yoongkit1014@thu.edu.tw
† These authors contributed equally to this work.
Received: 18 January 2025; Revised: 2 March 2025; Accepted: 6 March 2025; Published: 2 April 2025
Abstract: Microalgae are versatile platforms for producing biofuels and high-value metabolites, such as lipids, proteins, and carotenoids. Numerous stress strategies have been adopted to improve microalgal cultivation and biomolecule yield. This review examines how conventional stress factors (light and salinity) and unconventional treatments (electric field treatment) influence microalgal growth and metabolite accumulation. Light intensity, spectrum, and photoperiod significantly affect photosynthesis, biomass yield, and carotenoid biosynthesis, with moderate intensities found to enhance efficiency. However, excessive levels may induce photoinhibition. Salinity stress induces activation of antioxidant systems and lipid accumulation, optimizes biofuel properties. However, excessive high salinity can impair the growth of microalgae. In this review, we focused on the electric field treatment as a potential strategy for enhancing microalgal productivity, representing a major novelty of the study. Unlike traditional stress factors that primarily induce adaptive metabolic shifts, electric field treatment offers a unique and an understudied approach for modulating cellular physiology. Electric treatment technology offers an energy-efficient method for stimulating cell differentiation and enhancing lipid and pigment production while reducing environmental effects. Integrating these stress factors may be an attractive approach for controlling over microalgal metabolism, supporting sustainable and scalable biorefinery applications.
Keywords:
light modulation salinity stress pulsed electric field sustainable biofuel stress-induced pathways metabolic engineeringReferences
- Caroppo, C.; Pagliara, P. Microalgae: A Promising Future. Microorganisms 2022, 10, 1488. https://doi.org/10.3390/microorganisms10081488.
- Fernández, F.G.A.; Reis, A.; Wijffels, R.H.; et al. The role of microalgae in the bioeconomy. New Biotechnol. 2021, 61, 99–107. https://doi.org/10.1016/j.nbt.2020.11.011.
- Viegas, C.; Gouveia, L.; Gonçalves, M. Aquaculture wastewater treatment through microalgal. Biomass potential applications on animal feed, agriculture, and energy. J. Environ. Manag. 2021, 286, 112187. https://doi.org/10.1016/j.jenvman.2021.112187.
- Ramandani, A.A.; Lee, S.Y.; Jambrak, A.R.; et al. Synergizing food waste management and microalgae biorefinery for bioenergy production: Recent advance on direct and indirect conversion pathway. Process Biochem. 2025, 151, 14–26. https://doi.org/10.1016/j.procbio.2025.01.006.
- Goh, B.H.H.; Ong, H.C.; Cheah, M.Y.; et al. Sustainability of direct biodiesel synthesis from microalgae biomass: A critical review. Renew. Sustain. Energy Rev. 2019, 107, 59–74. https://doi.org/10.1016/j.rser.2019.02.012.
- Ashokkumar, V.; Flora, G.; Kumar, G.; et al. Cutting-edge advances in alga Botryococcus for eco-friendly biofuels and high-value bioproducts—A critical review. Algal Res. 2024, 83, 103676. https://doi.org/10.1016/j.algal.2024.103676.
- Barera, S.; Forlani, G. The role of proline in the adaptation of eukaryotic microalgae to environmental stress: An underestimated tool for the optimization of algal growth. J. Appl. Phycol. 2023, 35, 1635–1648. https://doi.org/10.1007/s10811-023-03017-9.
- Maltsev, Y.; Maltseva, K.; Kulikovskiy, M.; et al. Influence of light conditions on microalgae growth and content of lipids, carotenoids, and fatty acid composition. Biology 2021, 10, 1060. https://doi.org/10.3390/biology10101060.
- Begum, H.; Yusoff, F.M.D.; Banerjee, S.; et al. Availability and utilization of pigments from microalgae. Crit. Rev. Food Sci. Nutr. 2016, 56, 2209–2222. https://doi.org/10.1080/10408398.2013.764841.
- Singh, S.P.; Singh, P. Effect of temperature and light on the growth of algae species: A review. Renew. Sustain. Energy Rev. 2015, 50, 431–444. https://doi.org/10.1016/j.rser.2015.05.024.
- Fu, W.; Guomundsson, Ó.; Paglia, G.; et al. Enhancement of carotenoid biosynthesis in the green microalga Dunaliella salina with light-emitting diodes and adaptive laboratory evolution. Appl. Microbiol. Biotechnol. 2013, 97, 2395–2403. https://doi.org/10.1007/s00253-012-4502-5.
- Leong, Y.K.; Chang, J.S. Lutein biosynthesis from microalgae — Recent advances and circular economy. Environ. Technol. Innov. 2023, 30, 103097. https://doi.org/10.1016/j.eti.2023.103097.
- Saha, S.K.; Kazipet, N.; Murray, P. The carotenogenic Dunaliella salina CCAP 19/20 produces enhanced levels of carotenoid under specific nutrients limitation. BioMed Res. Int. 2018, 2018, 7532897. https://doi.org/10.1155/2018/7532897.
- Jahns, P.; Holzwarth, A.R. The role of the xanthophyll cycle and of lutein in photoprotection of photosystem II. Biochim. Et Biophys. Acta—Bioenerg. 2012, 1817, 182–193. https://doi.org/10.1016/j.bbabio.2011.04.012.
- Kuczynska, P.; Jemiola-Rzeminska, M.; Nowicka, B.; et al. The xanthophyll cycle in diatom Phaeodactylum tricornutum in response to light stress. Plant Physiol. Biochem. 2020, 152, 125–137. https://doi.org/10.1016/j.plaphy.2020.04.043.
- Schulze, P.S.C.; Barreira, L.A.; Pereira, H.G.C.; et al. Light emitting diodes (LEDs) applied to microalgal production. Trends Biotechnol. 2014, 32, 422–430. https://doi.org/https://doi.org/10.1016/j.tibtech.2014.06.001.
- Kou, Y.; Liu, M.; Sun, P.; et al. High light boosts salinity stress-induced biosynthesis of astaxanthin and lipids in the green alga Chromochloris zofingiensis. Algal Res. 2020, 50, 101976. https://doi.org/https://doi.org/10.1016/j.algal.2020.101976.
- Gouveia, L.; Neves, C.; Sebastião, D.; et al. Effect of light on the production of bioelectricity and added-value microalgae biomass in a Photosynthetic Alga Microbial Fuel Cell. Bioresour. Technol. 2014, 154, 171–177. https://doi.org/10.1016/j.biortech.2013.12.049.
- Zhang, Y.; Shi, M.; Mao, X.; et al. Time-resolved carotenoid profiling and transcriptomic analysis reveal mechanism of carotenogenesis for astaxanthin synthesis in the oleaginous green alga Chromochloris zofingiensis. Biotechnol. Biofuels 2019, 12, 287. https://doi.org/10.1186/s13068-019-1626-1.
- Kim, T.H.; Lee, Y.; Han, S.H.; et al. The effects of wavelength and wavelength mixing ratios on microalgae growth and nitrogen, phosphorus removal using Scenedesmus sp. for wastewater treatment. Bioresour. Technol. 2013, 130, 75–80. https://doi.org/10.1016/j.biortech.2012.11.134.
- Jayakumar, S.; Bhuyar, P.; Pugazhendhi, A.; et al. Effects of light intensity and nutrients on the lipid content of marine microalga (diatom) Amphiprora sp. for promising biodiesel production. Sci. Total Environ. 2021, 768, 145471. https://doi.org/10.1016/j.scitotenv.2021.145471.
- Han, W.; Jin, W.; Ding, W.; et al. Effects of nutrient composition, lighting conditions, and metal ions on the growth and lipid yield of the high-lipid-yielding microalgae (Chlorella pyrenoidosa) cultivated in municipal wastewater. J. Environ. Chem. Eng. 2021, 9, 106491. https://doi.org/10.1016/j.jece.2021.106491.
- Patel, A.K.; Vadrale, A.P.; Singhania, R.R.; et al. Enhanced mixotrophic production of lutein and lipid from potential microalgae isolate Chlorella sorokiniana C16. Bioresour. Technol. 2023, 386, 129477. https://doi.org/10.1016/j.biortech.2023.129477.
- Cheah, W.Y.; Show, P.L.; Juan, J.C.; et al. Enhancing biomass and lipid productions of microalgae in palm oil mill effluent using carbon and nutrient supplementation. Energy Convers. Manag. 2018, 164, 188–197. https://doi.org/10.1016/j.enconman.2018.02.094.
- Chang, W.; Li, Y.; Qu, Y.; et al. Mixotrophic cultivation of microalgae to enhance the biomass and lipid production with synergistic effect of red light and phytohormone IAA. Renew. Energy 2022, 187, 819–828. https://doi.org/10.1016/j.renene.2022.01.108.
- Felix, C.; Ubando, A.; Madrazo, C.; et al. Non-catalytic in-situ (trans)esterification of lipids in wet microalgae Chlorella vulgaris under subcritical conditions for the synthesis of fatty acid methyl esters. Appl. Energy 2019, 248, 526–537. https://doi.org/10.1016/j.apenergy.2019.04.149.
- Yen, S.W.; Nagarajan, D.; Chen, W.H.; et al. Fermentative production of astaxanthin from sorghum distillery residue by an indigenous Aurantiochytrium sp. CJ6 strain using a continuous-feeding fed-batch process. Bioresour. Technol. 2023, 376, 128817. https://doi.org/10.1016/j.biortech.2023.128817.
- Liu, M.; Chen, Y.; Zhu, C.; et al. Insight into the regulation mechanism of light spectra on astaxanthin biosynthesis in mixotrophic Chromochloris zofingiensis. Algal Res. 2024, 82, 103690. https://doi.org/10.1016/j.algal.2024.103690.
- Ma, R.; Tao, X.; Wang, B.; et al. Hyper-production of astaxanthin from Haematococcus pluvialis by a highly efficient nitrogen feeding strategy accompanied with high light induction. Algal Res. 2025, 85, 103865. https://doi.org/10.1016/j.algal.2024.103865.
- Cheirsilp, B.; Wantip, K.; Chai-issarapap, N.; et al. Enhanced production of astaxanthin and co-bioproducts from microalga Haematococcus sp. integrated with valorization of industrial wastewater under two-stage LED light illumination strategy. Environ. Technol. Innov. 2022, 28, 102620. https://doi.org/10.1016/j.eti.2022.102620.
- Zhang, H.; Tan, Y.; Zhu, R.; et al. Red light trade-off regulating the negative effects of nitrogen concentration to improve lipid and astaxanthin production in the filamentous green microalga Oedocladium carolinianum. Algal Res. 2024, 80, 03510. https://doi.org/10.1016/j.algal.2024.103510.
- Nur, M.M.A.; Liana, F.M.; Putri, A.N.L.D.; et al. Enhancing fucoxanthin production in Chaetoceros calcitrans under mixotrophic condition and LED wavelength shift strategy. Biocatal. Agric. Biotechnol. 2024, 57, 103120. https://doi.org/10.1016/j.bcab.2024.103120.
- Bo, Y.; Wang, S.; Ma, F.; et al. The influence of spermidine on the build-up of fucoxanthin in Isochrysis sp. acclimated to varying light intensities. Bioresour. Technol. 2023, 387, 129688. https://doi.org/10.1016/j.biortech.2023.129688.
- Zhang, H.; Gong, P.; Cai, Q.; et al. Maximizing fucoxanthin production in Odontella aurita by optimizing the ratio of red and blue light-emitting diodes in an auto-controlled internally illuminated photobioreactor. Bioresour. Technol. 2022, 344, 126260. https://doi.org/10.1016/j.biortech.2021.126260.
- Chen, C.Y.; Liu, P.Y.; Chang, Y.H.; et al. Optimizing cultivation strategies and scaling up for fucoxanthin production using Pavlova sp. Bioresour. Technol. 2024, 399, 130609. https://doi.org/10.1016/j.biortech.2024.130609.
- Weickert, S.; Schmid-Staiger, U.; Lewandowski, I. Influence of specific light availability and solvent on process economics—The production of fucoxanthin and eicosapentaenoic acid from P. tricornutum using flat-panel airlift photobioreactors with artificial light. Algal Res. 2023, 75, 103284. https://doi.org/10.1016/j.algal.2023.103284.
- Zhao, X.; Yan, J.; Yang, T.; et al. Exploring engineering reduced graphene oxide-titanium dioxide (rGO-TiO2) nanoparticles treatment to effectively enhance lutein biosynthesis with Chlorella sorokiniana F31 under different light intensity. Bioresour. Technol. 2022, 348, 126816. https://doi.org/10.1016/j.biortech.2022.126816.
- Zheng, H.; Wang, Y.; Li, S.; et al. Lutein production by microalgae using corn starch wastewater pretreated with rapid enzymatic hydrolysis. Bioresour. Technol. 2022, 352, 126940. https://doi.org/10.1016/j.biortech.2022.126940.
- Chen, J.H.; Kato, Y.; Matsuda, M.; et al. Lutein production with Chlorella sorokiniana MB-1-M12 using novel two-stage cultivation strategies—Metabolic analysis and process improvement. Bioresour. Technol. 2021, 334, 125200. https://doi.org/10.1016/j.biortech.2021.125200.
- Yu, H.Y.; Cho, D.H.; Seo, D.; et al. Microalga Chlorella sp. biomass containing high lutein prevents light-induced photooxidation and retinal degeneration in mice. Algal Res. 2024, 82, 103620. https://doi.org/10.1016/j.algal.2024.103620.
- Diaz-MacAdoo, D.; Nagai, S.; Mata, M.T.; et al. Comparative analysis of carotenoid synthesis and transcriptome of a microalga Chlorophyta MCH-35, potential lutein producer, in response to different quality light. Algal Res. 2023, 74, 103206. https://doi.org/10.1016/j.algal.2023.103206.
- Liu, Y.; Wei, D. Enhancing carbon dioxide fixation and co-production of protein and lutein in oleaginous Coccomyxa subellipsoidea by a stepwise light intensity and nutrients feeding strategy. Bioresour. Technol. 2023, 376, 128885. https://doi.org/10.1016/j.biortech.2023.128885.
- Li, J.; Zhao, X.; Chang, J.S.; et al. A two-stage culture strategy for Scenedesmus sp. FSP3 for CO2 fixation and the simultaneous production of lutein under light and salt stress. Molecules 2022, 27, 7497. https://doi.org/10.3390/molecules27217497.
- Bialevich, V.; Zachleder, V.; Bišová, K. The effect of variable light source and light intensity on the growth of three algal species. Cells 2022, 11, 1293. https://doi.org/10.3390/cells11081293.
- Difusa, A.; Talukdar, J.; Kalita, M.C.; et al. Effect of light intensity and pH condition on the growth, biomass and lipid content of microalgae Scenedesmus species. Biofuels 2015, 6, 37–44. https://doi.org/10.1080/17597269.2015.1045274.
- Gim, G.H.; Ryu, J.; Kim, M.J.; et al. Effects of carbon source and light intensity on the growth and total lipid production of three microalgae under different culture conditions. J. Ind. Microbiol. Biotechnol. 2016, 43, 605–616. https://doi.org/10.1007/s10295-016-1741-y.
- Cheirsilp, B.; Torpee, S. Enhanced growth and lipid production of microalgae under mixotrophic culture condition: Effect of light intensity, glucose concentration and fed-batch cultivation. Bioresour. Technol. 2012, 110, 510–516. https://doi.org/10.1016/j.biortech.2012.01.125.
- Ho, S.H.; Chen, C.Y.; Chang, J.S. Effect of light intensity and nitrogen starvation on CO2 fixation and lipid/carbohydrate production of an indigenous microalga Scenedesmus obliquus CNW-N. Bioresour. Technol. 2012, 113, 244–252. https://doi.org/10.1016/j.biortech.2011.11.133.
- Mahari, W.A.W.; Razali, W.A.W.; Khor, W.; et al. Light-emitting diodes (LEDs) for culturing microalgae and cyanobacteria. Chem. Eng. J. 2024, 485, 149619. https://doi.org/10.1016/j.cej.2024.149619.
- You, T.; Barnett, S.M. Effect of light quality on production of extracellular polysaccharides and growth rate of Porphyridium Cruentum. Biochem. Eng. J. 2004, 19, 251–258. https://doi.org/https://doi.org/10.1016/j.bej.2004.02.004.
- Abu-Ghosh, S.; Fixler, D.; Dubinsky, Z.; Iluz, D. Continuous background light significantly increases flashing-light enhancement of photosynthesis and growth of microalgae. Bioresour. Technol. 2015, 187, 144–148. https://doi.org/10.1016/j.biortech.2015.03.119.
- Vélez-Landa, L.; Hernández-De León, H.R.; Pérez-Luna, Y.D.C.; et al. Influence of light intensity and photoperiod on the photoautotrophic growth and lipid content of the microalgae Verrucodesmus verrucosus in a photobioreactor. Sustainability 2021, 13, 6606. https://doi.org/10.3390/su13126606.
- Chakravarty, S.; Mallick, N. Engineering a cultivation strategy for higher lipid accretion and biodiesel production by the marine microalga Picochlorum soloecismus. Sustain. Chem. Pharm. 2022, 26, 100635. https://doi.org/10.1016/j.scp.2022.100635.
- Anahas AM, P.; Prasannabalaji, N.; Muralitharan, G. Unlocking the potential of coal mine microalgae strains: Enhanced biodiesel production and CO₂ sequestration through cultivation optimization. Biomass Bioenergy 2025, 192, 107489. https://doi.org/10.1016/j.biombioe.2024.107489.
- Almutairi, A.W. Harnessing waste glycerol to mitigate salinity constraints in freshwater microalgae cultivation for enhanced biodiesel recovery. J. King Saud Univ.-Sci. 2024, 36, 103258. https://doi.org/10.1016/j.jksus.2024.103258.
- Sinetova, M.A.; Sidorov, R.A.; Medvedeva, A.A.; et al. Effect of salt stress on physiological parameters of microalgae Vischeria punctata strain IPPAS H-242, a superproducer of eicosapentaenoic acid. J. Biotechnol. 2021, 331, 63–73. https://doi.org/10.1016/j.jbiotec.2021.03.001.
- Liu, N.; Mou, Y.; Su, K.; et al. The effect of salinity stress on the growth and lipid accumulation of Scenedesmus quadricauda FACHB-1297 under xylose mixotrophic cultivation. Process Saf. Environ. Prot. 2022, 165, 887–894. https://doi.org/10.1016/j.psep.2022.07.051.
- Chauhan, A.J.; Patel, A.K.; Chen, C.W.; et al. Enhanced production of high-value polyunsaturated fatty acids (PUFAs) from potential thraustochytrid Aurantiochytrium sp. Bioresour. Technol. 2023, 370, 128536. https://doi.org/10.1016/j.biortech.2022.128536.
- Yang, Z.; Chen, J.; Tang, B.; et al. Metabolic interpretation of NaCl stress-induced lipid accumulation in microalgae for promising biodiesel production with saline wastewater. Chem. Eng. Sci. 2024, 284, 119447. https://doi.org/10.1016/j.ces.2023.119447.
- Sorokina, K.N.; Samoylova, Y.V.; Parmon, V.N. Comparative analysis of microalgae metabolism on BBM and municipal wastewater during salt induced lipid accumulation. Bioresour. Technol. Rep. 2020, 11, 100548. https://doi.org/10.1016/j.biteb.2020.100548.
- Anand, V.; Kashyap, M.; Sharma, M.P.; et al. Impact of hydrogen peroxide on microalgae cultivated in varying salt-nitrate-phosphate conditions. J. Environ. Chem. Eng. 2021, 9, 105814. https://doi.org/10.1016/j.jece.2021.105814.
- Chen, C.Y.; Lee, M.H.; Dong, C.D.; et al. Enhanced production of microalgal lipids using a heterotrophic marine microalga Thraustochytrium sp. BM2. Biochem. Eng. J. 2020, 154, 107429. https://doi.org/10.1016/j.bej.2019.107429.
- Chen, C.Y.; Lee, M.H.; Leong, Y.K.; et al. Biodiesel production from heterotrophic oleaginous microalga Thraustochytrium sp. BM2 with enhanced lipid accumulation using crude glycerol as alternative carbon source. Bioresour. Technol. 2020, 306, 123113. https://doi.org/10.1016/j.biortech.2020.123113.
- Zhao, Y.; Li, Q.; Gu, D.; et al. The synergistic effects of gamma-aminobutyric acid and salinity during the enhancement of microalgal lipid production in photobioreactors. Energy Convers. Manag. 2022, 267, 115928. https://doi.org/10.1016/j.enconman.2022.115928.
- Mandal, M.K.; Chaurasia, N. De novo transcriptomic analysis of Graesiella emersonii NC-M1 reveals differential genes expression in cell proliferation and lipid production under glucose and salt supplemented condition. Renew. Energy 2021, 179, 2004–2015. https://doi.org/10.1016/j.renene.2021.07.141.
- Parveen, A.; Rawat, J.; Bhatnagar, P.; et al. Enhanced production of high-value compounds from Chlorella sorokiniana by two-stage cultivation under red light and salinity stress. Biocatal. Agric. Biotechnol. 2024, 60, 103315. https://doi.org/10.1016/j.bcab.2024.103315.
- Ma, M.; Jiang, L.; He, Y.; et al. Multi-faceted effects of NaCl on salt-tolerant microalgae Golenkinia sp. SDEC-16. Bioresour. Technol. 2024, 406, 131016. https://doi.org/10.1016/j.biortech.2024.131016.
- Pereira, R.N.; Jaeschke, D.P.; Mercali, G.D.; et al. Impact of ultrasound and electric fields on microalgae growth: A comprehensive review. Braz. J. Chem. Eng. 2023, 40, 607–622. https://doi.org/10.1007/s43153-022-00281-z.
- La, H.J.; Choi, G.G.; Cho, C.; et al. Increased lipid productivity of Acutodesmus dimorphus using optimized pulsed electric field. J. Appl. Phycol. 2016, 28, 931–938. https://doi.org/10.1007/s10811-015-0674-6.
- Carullo, D.; Abera, B.D.; Casazza, A.A.; et al. Effect of pulsed electric fields and high pressure homogenization on the aqueous extraction of intracellular compounds from the microalgae Chlorella vulgaris. Algal Res. 2018, 31, 60–69. https://doi.org/10.1016/j.algal.2018.01.017.
- Haberkorn, I.; Buchmann, L.; Hiestand, M.; et al. Continuous nanosecond pulsed electric field treatments foster the upstream performance of Chlorella vulgaris-based biorefinery concepts. Bioresour. Technol. 2019, 293, 122029. https://doi.org/10.1016/j.biortech.2019.122029.
- Buchmann, L.; Böcker, L.; Frey, W.; et al. Energy input assessment for nanosecond pulsed electric field processing and its application in a case study with Chlorella vulgaris. Innov. Food Sci. Emerg. Technol. 2018, 47, 445–453. https://doi.org/10.1016/j.ifset.2018.04.013.
- Nezammahalleh, H.; Ghanati, F.; Adams, T.A.; et al. Effect of moderate static electric field on the growth and metabolism of Chlorella vulgaris. Bioresour. Technol. 2016, 218, 700–711. https://doi.org/10.1016/j.biortech.2016.07.018.
- Canelli, G.; Kuster, I.; Jaquenod, L.; et al. Pulsed electric field treatment enhances lipid bioaccessibility while preserving oxidative stability in Chlorella vulgaris. Innov. Food Sci. Emerg. Technol. 2022, 75, 102897. https://doi.org/10.1016/j.ifset.2021.102897.
- Kim, J.Y.; Lee, C.; Jeon, M.S.; et al. Enhancement of microalga Haematococcus pluvialis growth and astaxanthin production by electrical treatment. Bioresour. Technol. 2018, 268, 815–819. https://doi.org/10.1016/j.biortech.2018.08.014.
- Barreiros, M.; Maciel, F.; Pereira, R.S.; et al. Electric fields to support microalgae growth with a differentiated biochemical composition. Innov. Food Sci. Emerg. Technol. 2024, 97, 103829. https://doi.org/10.1016/j.ifset.2024.103829.
- Choi, S.A.; Lee, S.Y.; Lee, J.; et al. Rapid induction of edible lipids in Chlorella by mild electric stimulation. Bioresour. Technol. 2019, 292. https://doi.org/10.1016/j.biortech.2019.121950.
- Ramos, M.; Dias, A.P.S.; Puna, J.F.; et al. Biodiesel production processes and sustainable raw materials. Energies 2019, 12, 4408. https://doi.org/10.3390/en12234408.
- Santos, S.; Puna, J.; Gomes, J. A review on bio-based catalysts (immobilized enzymes) used for biodiesel production. Energies 2020, 13, 3013. https://doi.org/10.3390/en13113013.