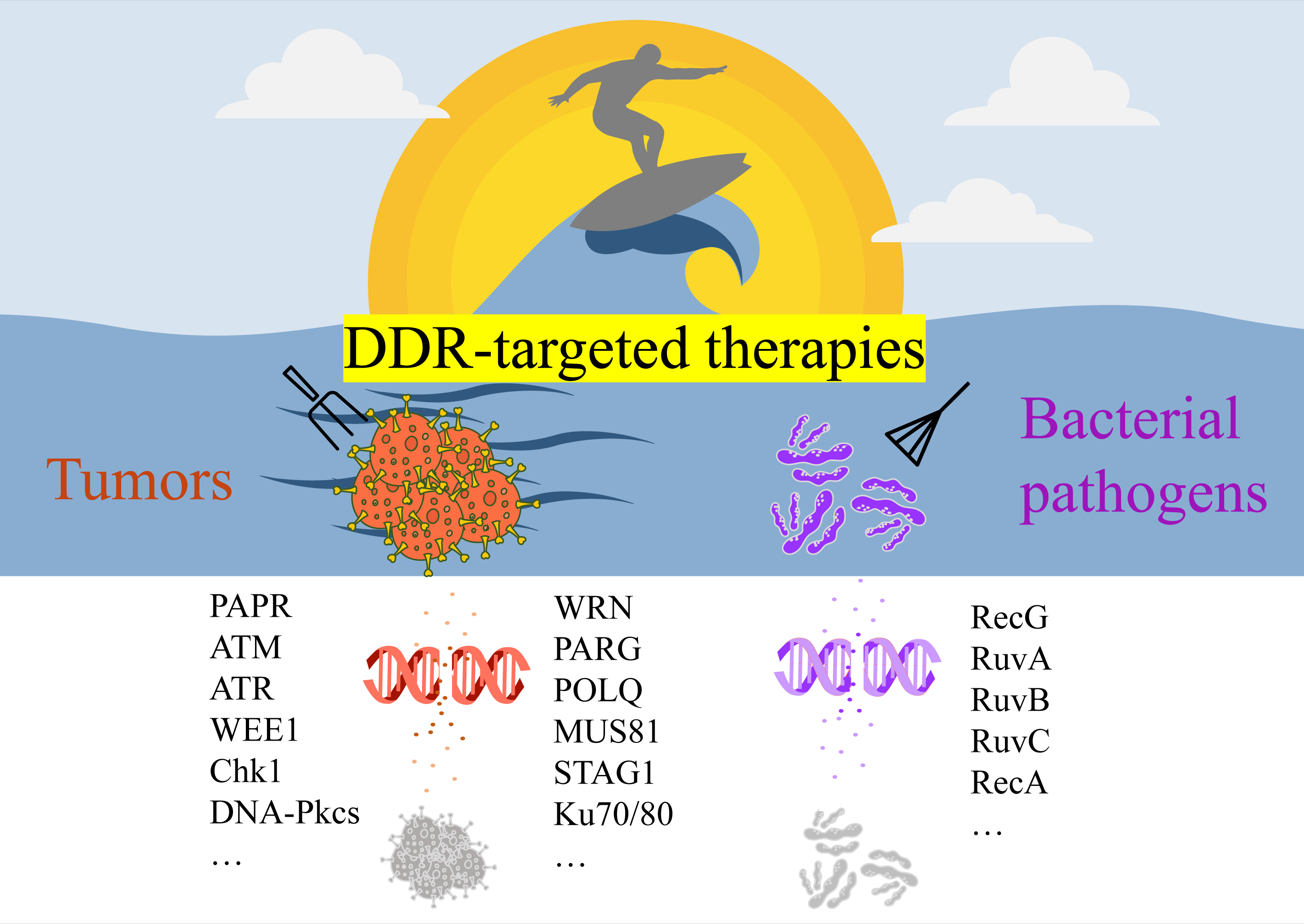
Downloads
Download


This work is licensed under a Creative Commons Attribution 4.0 International License.
Review
Targeting Cellular DNA Damage Response in Cancer and Bacterial Infections: Current Progress, Challenges, and Opportunities
Ranxun Lin 1, Xu Zhang 1, Qinwei Zhu 1, Xuening Chen 1, Lin Dai 1, Longheng Li 1, Zuoan Li 2, and Zhonghui Lin 1,*
1 College of Chemistry, Fuzhou University, Fuzhou 350108, China
2 Shengli Clinical Medical College of Fujian Medical University, Department of Emergency, Fujian Provincial Hospital, Fuzhou University Affiliated Provincial Hospital, Fujian Provincial Key Laboratory of Emergency Medicine, Fuzhou 350108, China
* Correspondence: zhonghui.lin@fzu.edu.cn
Received: 20 November 2024; Revised: 30 December 2024; Accepted: 21 February 2025; Published: 3 April 2025
Abstract: Effective cancer treatment remains challenging due to the genomic instability of tumors and the frequent emergence of resistance. Traditional approaches such as radiotherapy, chemotherapy, and immunotherapy face limitations in addressing tumor heterogeneity and resistance mechanisms. Targeting the DNA damage response (DDR) pathway has emerged as an innovative strategy, either as monotherapy or in combination with conventional treatments. DDR-targeted therapies, including poly-ADP-ribose polymerase (PARP) inhibitors, have shown promise in reducing tumor growth and enhancing patient outcomes. Emerging targets such as ATM, ATR, CHK1/2, WRN, and PARG, coupled with cutting-edge technologies like CRISPR and proteolysis-targeting chimeras (PROTACs), have opened new avenues for precise and effective cancer treatment. Furthermore, combining DDR inhibitors with established therapies, such as immune checkpoint inhibitors, has demonstrated synergistic benefits, improving therapeutic efficacy and overcoming resistance. Beyond cancer, DDR inhibitors also offer the potential to combat bacterial pathogens by exploiting vulnerabilities in microbial DNA repair systems. This review focuses on the major advantages, challenges, and future directions of DDR-targeted therapies in cancer and bacterial infections. We also discuss the integration of these therapies with traditional approaches, highlighting their potential to enhance therapeutic outcomes across diverse applications.
Keywords:
DNA damage response drug targets caner therapy bacterial infection treatment challenges and opportunitiesReferences
- Mole, R.H.; Temple, D.M. The DNA Content of the Small Intestine as a Quantitative Measure of Damage and Recovery after Whole Body Irradiation. Int. J. Radiat. Biol. Relat. Stud. Phys. Chem. Med. 1959, 1, 28–42. https://doi.org/10.1080/09553005914550061.
- Lerman, L.S.; Tolmach, L.J. Genetic Transformation II. The Significance of Damage to the DNA Molecule. Biochim. Biophys. Acta 1959, 33, 371–387. https://doi.org/10.1016/0006-3002(59)90127-1.
- Lett, J.T.; Stacey, K.A. The Relationship between Molecular Weight and Viscosity as a Criterion of Damage in Deoxyribonucleic Acid (DNA). Makromol. Chem. 1960, 38, 204–211. https://doi.org/10.1002/macp.1960.020380117.
- De Bont, R.; van Larebeke, N. Endogenous DNA Damage in Humans: A Review of Quantitative Data. Mutagenesis 2004, 19, 169–185. https://doi.org/10.1093/mutage/geh025.
- Ames, B.N. Endogenous DNA Damage as Related to Cancer and Aging. Mutat. Res. Mol. Mech. Mutagen. 1989, 214, 41–46. https://doi.org/10.1016/0027-5107(89)90196-6.
- Barbin, A.; Ohgaki, H.; Nakamura, J.; Kurrer, M.O.; Kleihues, P.; Swenberg, J.A. Endogenous Deoxyribonucleic Acid (DNA) Damage in Human Tissues: A Comparison of Ethenobases with Aldehydic DNA Lesions. Cancer Epidemiol. Biomark. Prev. 2003, 12, 1241–1247.
- Yokoya, A.; Obata, Y. Core Level Ionization or Excitation and Auger Relaxation Induce Clustered DNA Damage. In The Enzymes; Elsevier: Amsterdam, The Netherlands, 2022; Volume 51, pp. 79–100. https://doi.org/10.1016/bs.enz.2022.08.006.
- Stingele, J.; Bellelli, R.; Boulton, S.J. Mechanisms of DNA–Protein Crosslink Repair. Nat. Rev. Mol. Cell Biol. 2017, 18, 563–573. https://doi.org/10.1038/nrm.2017.56.
- Thomas, C.S.; Pollard, H.C.; Razskazovskiy, Y.; Roginskaya, M. Sources of 2,5-Diaminoimidazolone Lesions in DNA Damage Initiated by Hydroxyl Radical Attack. Free Radic. Res. 2020, 54, 517–524. https://doi.org/10.1080/10715762.2020.1808632.
- Ciccia, A.; Elledge, S.J. The DNA Damage Response: Making It Safe to Play with Knives. Mol. Cell 2010, 40, 179–204. https://doi.org/10.1016/j.molcel.2010.09.019.
- Di Domenico, E.G.; Romano, E.; Del Porto, P.; Ascenzioni, F. Multifunctional Role of ATM/Tel1 Kinase in Genome Stability: From the DNA Damage Response to Telomere Maintenance. BioMed Res. Int. 2014, 2014, 787404. https://doi.org/10.1155/2014/787404.
- Bartkova, J.; Hořejší, Z.; Koed, K.; Krämer, A.; Tort, F.; Zieger, K.; Guldberg, P.; Sehested, M.; Nesland, J.M.; Lukas, C.; et al. DNA Damage Response as a Candidate Anti-Cancer Barrier in Early Human Tumorigenesis. Nature 2005, 434, 864–870. https://doi.org/10.1038/nature03482.
- Jiang, A.; Song, J.; Fang, X.; Fang, Y.; Wang, Z.; Liu, B.; Wu, Z.; Qu, L.; Luo, P.; Wang, L. A Novel Thinking: DDR Axis Refines the Classification of ccRCC with Distinctive Prognosis, Multi Omics Landscape and Management Strategy. Front. Public Health 2022, 10, 1029509. https://doi.org/10.3389/fpubh.2022.1029509.
- Lord, C.J.; Ashworth, A. BRCAness Revisited. Nat. Rev. Cancer 2016, 16, 110–120. https://doi.org/10.1038/nrc.2015.21.
- Lord, C.J.; Ashworth, A. PARP Inhibitors: Synthetic Lethality in the Clinic. Science 2017, 355, 1152–1158. https://doi.org/10.1126/science.aam7344.
- Shor, E.; Perlin, D.S. DNA Damage Response of Major Fungal Pathogen Candida Glabrata Offers Clues to Explain Its Genetic Diversity. Curr. Genet. 2021, 67, 439–445. https://doi.org/10.1007/s00294-021-01162-7.
- Samba-Louaka, A.; Pereira, J.M.; Nahori, M.-A.; Villiers, V.; Deriano, L.; Hamon, M.A.; Cossart, P. Listeria Monocytogenes Dampens the DNA Damage Response. PLoS Pathog. 2014, 10, e1004470. https://doi.org/10.1371/journal.ppat.1004470.
- Shen, L.L.; Baranowski, J.; Fostel, J.; Montgomery, D.A.; Lartey, P.A. DNA Topoisomerases from Pathogenic Fungi: Targets for the Discovery of Antifungal Drugs. Antimicrob. Agents Chemother. 1992, 36, 2778–2784. https://doi.org/10.1128/AAC.36.12.2778.
- Jackson, S.P.; Bartek, J. The DNA-Damage Response in Human Biology and Disease. Nature 2009, 461, 1071–1078. https://doi.org/10.1038/nature08467.
- Ward, I.M.; Chen, J. Histone H2AX Is Phosphorylated in an ATR-Dependent Manner in Response to Replicational Stress. J. Biol. Chem. 2001, 276, 47759–47762. https://doi.org/10.1074/jbc.C100569200.
- Chanoux, R.A.; Yin, B.; Urtishak, K.A.; Asare, A.; Bassing, C.H.; Brown, E.J. ATR and H2AX Cooperate in Maintaining Genome Stability under Replication Stress. J. Biol. Chem. 2009, 284, 5994–6003. https://doi.org/10.1074/jbc.M806739200.
- Burma, S.; Chen, B.P.; Murphy, M.; Kurimasa, A.; Chen, D.J. ATM Phosphorylates Histone H2AX in Response to DNA Double-Strand Breaks. J. Biol. Chem. 2001, 276, 42462–42467. https://doi.org/10.1074/jbc.C100466200.
- Guo, X.; Deng, Y.; Lin, Y.; Cosme-Blanco, W.; Chan, S.; He, H.; Yuan, G.; Brown, E.J.; Chang, S. Dysfunctional Telomeres Activate an ATM-ATR-dependent DNA Damage Response to Suppress Tumorigenesis. EMBO J. 2007, 26, 4709–4719. https://doi.org/10.1038/sj.emboj.7601893.
- Roy, R.; Chun, J.; Powell, S.N. BRCA1 and BRCA2: Different Roles in a Common Pathway of Genome Protection. Nat. Rev. Cancer 2012, 12, 68–78. https://doi.org/10.1038/nrc3181.
- Panier, S.; Boulton, S.J. Double-Strand Break Repair: 53BP1 Comes into Focus. Nat. Rev. Mol. Cell Biol. 2014, 15, 7–18. https://doi.org/10.1038/nrm3719.
- Mahaney, B.L.; Meek, K.; Lees-Miller, S.P. Repair of Ionizing Radiation-Induced DNA Double-Strand Breaks by Non-Homologous End-Joining. Biochem. J. 2009, 417, 639–650. https://doi.org/10.1042/BJ20080413.
- Schreiber, V.; Dantzer, F.; Ame, J.-C.; de Murcia, G. Poly(ADP-Ribose): Novel Functions for an Old Molecule. Nat. Rev. Mol. Cell Biol. 2006, 7, 517–528. https://doi.org/10.1038/nrm1963.
- Meek, K.; Gupta, S.; Ramsden, D.A.; Lees-Miller, S.P. The DNA-Dependent Protein Kinase: The Director at the End. Immunol. Rev. 2004, 200, 132–141. https://doi.org/10.1111/j.0105-2896.2004.00162.x.
- Smith, J.; Mun Tho, L.; Xu, N.; Gillespie, D.A. Chapter 3–The ATM–Chk2 and ATR–Chk1 Pathways in DNA Damage Signaling and Cancer. In Advances in Cancer Research; Vande Woude, G.F., Klein, G., Eds.; Academic Press: Cambridge, MA, USA, 2010; Volume 108, pp. 73–112. https://doi.org/10.1016/B978-0-12-380888-2.00003-0.
- Stolz, A.; Ertych, N.; Bastians, H. Tumor Suppressor CHK2: Regulator of DNA Damage Response and Mediator of Chromosomal Stability. Clin. Cancer Res. 2011, 17, 401–405. https://doi.org/10.1158/1078-0432.CCR-10-1215.
- Vousden, K.H.; Lane, D.P. P53 in Health and Disease. Nat. Rev. Mol. Cell Biol. 2007, 8, 275–283. https://doi.org/10.1038/nrm2147.
- Matada, G.S.P.; Das, A.; Dhiwar, P.S.; Ghara, A. DDR1 and DDR2: A Review on Signaling Pathway and Small Molecule Inhibitors as an Anticancer Agent. Med. Chem. Res. 2021, 30, 535–551. https://doi.org/10.1007/s00044-020-02694-2.
- Trono, P.; Ottavi, F.; Rosano’, L. Novel Insights into the Role of Discoidin Domain Receptor 2 (DDR2) in Cancer Progression: A New Avenue of Therapeutic Intervention. Matrix Biol. 2024, 125, 31–39. https://doi.org/10.1016/j.matbio.2023.12.003.
- Deeks, E.D. Olaparib: First Global Approval. Drugs 2015, 75, 231–240. https://doi.org/10.1007/s40265-015-0345-6.
- Lee, A. Fuzuloparib: First Approval. Drugs 2021, 81, 1221–1226. https://doi.org/10.1007/s40265-021-01541-x.
- Markham, A. Pamiparib: First Approval. Drugs 2021, 81, 1343–1348. https://doi.org/10.1007/s40265-021-01552-8.
- U.S. FDA Approves AKEEGATM (Niraparib and Abiraterone Acetate), the First-And-Only Dual Action Tablet for the Treatment of Patients with BRCA-Positive Metastatic Castration-Resistant Prostate Cancer. JNJ.com. Available online: https://www.jnj.com/media-center/press-releases/u-s-fda-approves-akeega-niraparib-and-abiraterone-acetate-the-first-and-only-dual-action-tablet-for-the-treatment-of-patients-with-brca-positive-metastatic-castration-resistant-prostate-cancer (accessed on 5 November 2024).
- Wu, X.; Liu, J.; Wang, J.; Wang, L.; Lin, Z.; Wang, X.; Zhu, J.; Kong, B.; Fei, J.; Tang, Y.; et al. Senaparib as First-Line Maintenance Therapy in Advanced Ovarian Cancer: A Randomized Phase 3 Trial. Nat. Med. 2024, 30, 1612–1621. https://doi.org/10.1038/s41591-024-03003-9.
- Ma, L.; Chen, W.; Yang, M.; Ha, S.; Xiong, S.; Zhu, J.; Xiang, H.; Luo, G. Discovery and Proof of Concept of Potent Dual Polθ/PARP Inhibitors for Efficient Treatment of Homologous Recombination-Deficient Tumors. J. Med. Chem. 2024, 67, 3606–3625. https://doi.org/10.1021/acs.jmedchem.3c02096.
- Gao, Y.; Duan, J.-L.; Wang, C.-C.; Yuan, Y.; Zhang, P.; Wang, Z.-H.; Sun, B.; Zhou, J.; Du, X.; Dang, X.; et al. Novel Bifunctional Conjugates Targeting PD-L1/PARP7 as Dual Immunotherapy for Potential Cancer Treatment. J. Med. Chem. 2024, 67, 10848–10874. https://doi.org/10.1021/acs.jmedchem.4c00296.
- Hickson, I.D. RecQ Helicases: Caretakers of the Genome. Nat. Rev. Cancer 2003, 3, 169–178. https://doi.org/10.1038/nrc1012.
- Tadokoro, T.; Kulikowicz, T.; Dawut, L.; Croteau, D.L.; Bohr, V.A. DNA Binding Residues in the RQC Domain of Werner Protein Are Critical for Its Catalytic Activities. Aging 2012, 4, 417–429. https://doi.org/10.18632/aging.100463.
- Chen, L.; Huang, S.; Lee, L.; Davalos, A.; Schiestl, R.H.; Campisi, J.; Oshima, J. WRN, the Protein Deficient in Werner Syndrome, Plays a Critical Structural Role in Optimizing DNA Repair. Aging Cell 2003, 2, 191–199. https://doi.org/10.1046/j.1474-9728.2003.00052.x.
- Picco, G.; Cattaneo, C.M.; van Vliet, E.J.; Crisafulli, G.; Rospo, G.; Consonni, S.; Vieira, S.F.; Rodríguez, I.S.; Cancelliere, C.; Banerjee, R.; et al. Werner Helicase Is a Synthetic-Lethal Vulnerability in Mismatch Repair–Deficient Colorectal Cancer Refractory to Targeted Therapies, Chemotherapy, and Immunotherapy. Cancer Discov. 2021, 11, 1923–1937. https://doi.org/10.1158/2159-8290.CD-20-1508.
- Ferretti, S.; Hamon, J.; de Kanter, R.; Scheufler, C.; Andraos-Rey, R.; Barbe, S.; Bechter, E.; Blank, J.; Bordas, V.; Dammassa, E.; et al. Discovery of WRN Inhibitor HRO761 with Synthetic Lethality in MSI Cancers. Nature 2024, 629, 443. https://doi.org/10.1038/s41586-024-07350-y.
- Matthews, J. AHR Toxicity and Signaling: Role of TIPARP and ADP-Ribosylation. Curr. Opin. Toxicol. 2017, 2, 50–57. https://doi.org/10.1016/j.cotox.2017.01.013.
- Bindesbøll, C.; Tan, S.; Bott, D.; Cho, T.; Tamblyn, L.; MacPherson, L.; Grønning-Wang, L.; Nebb, H.I.; Matthews, J. TCDD-Inducible Poly-ADP-Ribose Polymerase (TIPARP/PARP7) Mono-ADP-Ribosylates and Co-Activates Liver X Receptors. Biochem. J. 2016, 473, 899–910. https://doi.org/10.1042/BJ20151077.
- Rasmussen, M.; Tan, S.; Somisetty, V.S.; Hutin, D.; Olafsen, N.E.; Moen, A.; Anonsen, J.H.; Grant, D.M.; Matthews, J. PARP7 and Mono-ADP-Ribosylation Negatively Regulate Estrogen Receptor α Signaling in Human Breast Cancer Cells. Cells 2021, 10, 623. https://doi.org/10.3390/cells10030623.
- Gozgit, J.M.; Vasbinder, M.M.; Abo, R.P.; Kunii, K.; Kuplast-Barr, K.G.; Gui, B.; Lu, A.Z.; Molina, J.R.; Minissale, E.; Swinger, K.K.; et al. PARP7 Negatively Regulates the Type I Interferon Response in Cancer Cells and Its Inhibition Triggers Antitumor Immunity. Cancer Cell 2021, 39, 1214–1226.e10. https://doi.org/10.1016/j.ccell.2021.06.018.
- Spirtos, A.N.; Aljardali, M.W.; Challa, S.; Koul, S.; Lea, J.S.; Kraus, W.L.; Camacho, C.V. RBN-2397, a PARP7 Inhibitor, Synergizes with Paclitaxel to Inhibit Proliferation and Migration of Ovarian Cancer Cells. bioRxiv 2024. https://doi.org/10.1101/2024.08.20.608802.
- Yap, T.A.; Cervantes, A.; Falchook, G.S.; Patel, M.R.; Juric, D.; Waqar, S.N.; Schenk, E.L.; Shapiro, G.; Boni, V.; Perez, C.A.; et al. Abstract CT109: First-in-Class First-in-Human Phase 1 Trial and Translational Study of the Mono(ADP-Ribose) Polymerase-7 (PARP7) Inhibitor RBN-2397 in Patients with Selected Advanced Solid Tumors. Cancer Res. 2023, 83, CT109. https://doi.org/10.1158/1538-7445.AM2023-CT109.
- Kang, D.; Wang, Y.; Sun, X.; Yan, M.; Li, H.; Chen, M.; Lin, Y.; Long, W. Abstract 4535: JAB-26766: A Small-Molecule, Orally Bioavailable PARP7 Inhibitor with High Potency and Selectivity. Cancer Res. 2024, 84 (Suppl. S6), 4535. https://doi.org/10.1158/1538-7445.AM2024-4535.
- Koh, D.W.; Lawler, A.M.; Poitras, M.F.; Sasaki, M.; Wattler, S.; Nehls, M.C.; Stöger, T.; Poirier, G.G.; Dawson, V.L.; Dawson, T.M. Failure to Degrade Poly(ADP-Ribose) Causes Increased Sensitivity to Cytotoxicity and Early Embryonic Lethality. Proc. Natl. Acad. Sci. USA 2004, 101, 17699–17704. https://doi.org/10.1073/pnas.0406182101.
- IDEAYA Announces Phase 1 Expansion and Preliminary Clinical Proof-of-Concept for Potential First-in-Class PARG Inhibitor IDE161 in HRD Solid Tumors. Investor Relations | IDEAYA Biosciences. Available online: https://ir.ideayabio.com/2023-09-11-IDEAYA-Announces-Phase-1-Expansion-and-Preliminary-Clinical-Proof-of-Concept-for-Potential-First-in-Class-PARG-Inhibitor-IDE161-in-HRD-Solid-Tumors (accessed on 16 November 2024).
- A Phase 1 Study of ETX-19477 in People with Advanced Solid Tumors | Memorial Sloan Kettering Cancer Center. Available online: https://www.mskcc.org/cancer-care/clinical-trials/24-224 (accessed on 16 November 2024).
- Nijman, S.M.B.; Huang, T.T.; Dirac, A.M.G.; Brummelkamp, T.R.; Kerkhoven, R.M.; D’Andrea, A.D.; Bernards, R. The Deubiquitinating Enzyme USP1 Regulates the Fanconi Anemia Pathway. Mol. Cell 2005, 17, 331–339. https://doi.org/10.1016/j.molcel.2005.01.008.
- Huang, T.T.; Nijman, S.M.B.; Mirchandani, K.D.; Galardy, P.J.; Cohn, M.A.; Haas, W.; Gygi, S.P.; Ploegh, H.L.; Bernards, R.; D’Andrea, A.D. Regulation of Monoubiquitinated PCNA by DUB Autocleavage. Nat. Cell Biol. 2006, 8, 341–347. https://doi.org/10.1038/ncb1378.
- Oestergaard, V.H.; Langevin, F.; Kuiken, H.J.; Pace, P.; Niedzwiedz, W.; Simpson, L.J.; Ohzeki, M.; Takata, M.; Sale, J.E.; Patel, K.J. Deubiquitination of FANCD2 Is Required for DNA Crosslink Repair. Mol. Cell 2007, 28, 798–809. https://doi.org/10.1016/j.molcel.2007.09.020.
- Williams, S.A.; Maecker, H.L.; French, D.M.; Liu, J.; Gregg, A.; Silverstein, L.B.; Cao, T.C.; Carano, R.A.D.; Dixit, V.M. USP1 Deubiquitinates ID Proteins to Preserve a Mesenchymal Stem Cell Program in Osteosarcoma. Cell 2011, 146, 918–930. https://doi.org/10.1016/j.cell.2011.07.040.
- Mistry, H.; Hsieh, G.; Buhrlage, S.J.; Huang, M.; Park, E.; Cuny, G.D.; Galinsky, I.; Stone, R.M.; Gray, N.S.; D’Andrea, A.D.; et al. Small Molecule Inhibitors of USP1 Target ID1 Degradation in Leukemic Cells. Mol. Cancer Ther. 2013, 12, 2651. https://doi.org/10.1158/1535-7163.MCT-13-0103-T.
- Liang, Q.; Dexheimer, T.S.; Zhang, P.; Rosenthal, A.S.; Villamil, M.A.; You, C.; Zhang, Q.; Chen, J.; Ott, C.A.; Sun, H.; et al. A Selective USP1-UAF1 Inhibitor Links Deubiquitination to DNA Damage Responses. Nat. Chem. Biol. 2014, 10, 298. https://doi.org/10.1038/nchembio.1455.
- Schrempf, A.; Slyskova, J.; Loizou, J.I. Targeting the DNA Repair Enzyme Polymerase θ in Cancer Therapy. Trends Cancer 2021, 7, 98–111. https://doi.org/10.1016/j.trecan.2020.09.007.
- Lemée, F.; Bergoglio, V.; Fernandez-Vidal, A.; Machado-Silva, A.; Pillaire, M.-J.; Bieth, A.; Gentil, C.; Baker, L.; Martin, A.-L.; Leduc, C.; et al. DNA Polymerase θ Up-Regulation Is Associated with Poor Survival in Breast Cancer, Perturbs DNA Replication, and Promotes Genetic Instability. Proc. Natl. Acad. Sci. USA 2010, 107, 13390–13395. https://doi.org/10.1073/pnas.0910759107.
- Ceccaldi, R.; Liu, J.C.; Amunugama, R.; Hajdu, I.; Primack, B.; Petalcorin, M.I.R.; O’Connor, K.W.; Konstantinopoulos, P.A.; Elledge, S.J.; Boulton, S.J.; et al. Homologous-Recombination-Deficient Tumours Are Dependent on Polθ-Mediated Repair. Nature 2015, 518, 258–262. https://doi.org/10.1038/nature14184.
- Oh, G.; Wang, A.; Wang, L.; Li, J.; Werba, G.; Weissinger, D.; Zhao, E.; Dhara, S.; Hernandez, R.E.; Ackermann, A.; et al. POLQ Inhibition Elicits an Immune Response in Homologous Recombination–Deficient Pancreatic Adenocarcinoma via cGAS/STING Signaling. J. Clin. Investig. 2023, 133, e165934. https://doi.org/10.1172/JCI165934.
- Artios Doses First Patient in Phase 1/2a Study of Polθ Inhibitor ART4215-Artios Pharma. Available online: https://www.artios.com/press-release/artios-doses-first-patient-in-phase-1-2a-study-of-pol%ce%b8-inhibitor-art4215/ (accessed on 17 November 2024).
- McPherson, J.P.; Lemmers, B.; Chahwan, R.; Pamidi, A.; Migon, E.; Matysiak-Zablocki, E.; Moynahan, M.E.; Essers, J.; Hanada, K.; Poonepalli, A.; et al. Involvement of Mammalian Mus81 in Genome Integrity and Tumor Suppression. Science 2004, 304, 1822–1826. https://doi.org/10.1126/science.1094557.
- Wyatt, H.D.M.; Sarbajna, S.; Matos, J.; West, S.C. Coordinated Actions of SLX1-SLX4 and MUS81-EME1 for Holliday Junction Resolution in Human Cells. Mol. Cell 2013, 52, 234–247. https://doi.org/10.1016/j.molcel.2013.08.035.
- Lai, X.; Broderick, R.; Bergoglio, V.; Zimmer, J.; Badie, S.; Niedzwiedz, W.; Hoffmann, J.-S.; Tarsounas, M. MUS81 Nuclease Activity Is Essential for Replication Stress Tolerance and Chromosome Segregation in BRCA2-Deficient Cells. Nat. Commun. 2017, 8, 15983. https://doi.org/10.1038/ncomms15983.
- Yin, Y.; Liu, W.; Shen, Q.; Zhang, P.; Wang, L.; Tao, R.; Li, H.; Ma, X.; Zeng, X.; Cheong, J.-H.; et al. The DNA Endonuclease Mus81 Regulates ZEB1 Expression and Serves as a Target of BET4 Inhibitors in Gastric Cancer. Mol. Cancer Ther. 2019, 18, 1439–1450. https://doi.org/10.1158/1535-7163.MCT-18-0833.
- Zhong, A.; Zhang, H.; Xie, S.; Deng, M.; Zheng, H.; Wang, Y.; Chen, M.; Lu, R.; Guo, L. Inhibition of MUS81 Improves the Chemical Sensitivity of Olaparib by Regulating MCM2 in Epithelial Ovarian Cancer. Oncol. Rep. 2018, 39, 1747–1756. https://doi.org/10.3892/or.2018.6229.
- Zhang, X.; Chen, X.; Lu, L.; Fang, Q.; Liu, C.; Lin, Z. Identification of Small-Molecule Inhibitors of Human MUS81-EME1/2 by FRET-Based High-Throughput Screening. Bioorg. Med. Chem. 2023, 90, 117383. https://doi.org/10.1016/j.bmc.2023.117383.
- Beskow, C.; Skikuniene, J.; Holgersson, Å.; Nilsson, B.; Lewensohn, R.; Kanter, L.; Viktorsson, K. Radioresistant Cervical Cancer Shows Upregulation of the NHEJ Proteins DNA-PKcs, Ku70 and Ku86. Br. J. Cancer 2009, 101, 816–821. https://doi.org/10.1038/sj.bjc.6605201.
- Ihara, M.; Ashizawa, K.; Shichijo, K.; Kudo, T. Expression of the DNA-Dependent Protein Kinase Catalytic Subunit Is Associated with the Radiosensitivity of Human Thyroid Cancer Cell Lines. J. Radiat. Res. 2019, 60, 171–177. https://doi.org/10.1093/jrr/rry097.
- Ramos, H.R.; López, L.E.; Castro, W.Q.; Serra, C.M. High-Sensitivity Cardiac Troponins: Sex-Specific Values in Clinical Practice. Precision or Confusion? Hellenic J. Cardiol. 2019, 60, 171–177. https://doi.org/10.1016/j.hjc.2019.02.005.
- Lee, S.; Cho, K.-J.; Park, J.; Kim, S.Y.; Nam, S.Y.; Lee, B.-J.; Kim, S.-B.; Choi, S.-H.; Kim, J.H.; Ahn, S.D.; et al. Expressions of Ku70 and DNA-PKcs as Prognostic Indicators of Local Control in Nasopharyngeal Carcinoma. Int. J. Radiat. Oncol. 2005, 62, 1451–1457. https://doi.org/10.1016/j.ijrobp.2004.12.049.
- Hayashi, J.; Sakata, K.-I.; Someya, M.; Matsumoto, Y.; Satoh, M.; Nakata, K.; Hori, M.; Takagi, M.; Kondoh, A.; Himi, T.; et al. Analysis and Results of Ku and XRCC4 Expression in Hypopharyngeal Cancer Tissues Treated with Chemoradiotherapy. Oncol. Lett. 2012, 4, 151–155. https://doi.org/10.3892/ol.2012.674.
- Chen, X.; Chen, C.; Luo, C.; Liu, J.; Lin, Z. Discovery of UMI-77 as a Novel Ku70/80 Inhibitor Sensitizing Cancer Cells to DNA Damaging Agents in Vitro and in Vivo. Eur. J. Pharmacol. 2024, 975, 176647. https://doi.org/10.1016/j.ejphar.2024.176647.
- Zhou, J.; Nie, R.; He, Z.; Cai, X.; Chen, J.; Lin, W.; Yin, Y.; Xiang, Z.; Zhu, T.; Xie, J.; et al. STAG2 Regulates Homologous Recombination Repair and Sensitivity to ATM Inhibition. Adv. Sci. 2023, 10, 2302494. https://doi.org/10.1002/advs.202302494.
- Lawrence, M.S.; Stojanov, P.; Mermel, C.H.; Robinson, J.T.; Garraway, L.A.; Golub, T.R.; Meyerson, M.; Gabriel, S.B.; Lander, E.S.; Getz, G. Discovery and Saturation Analysis of Cancer Genes across 21 Tumour Types. Nature 2014, 505, 495–501. https://doi.org/10.1038/nature12912.
- Van Der Lelij, P.; Lieb, S.; Jude, J.; Wutz, G.; Santos, C.P.; Falkenberg, K.; Schlattl, A.; Ban, J.; Schwentner, R.; Hoffmann, T.; et al. Synthetic Lethality between the Cohesin Subunits STAG1 and STAG2 in Diverse Cancer Contexts. Elife 2017, 6, e26980. https://doi.org/10.1101/155309.
- Benedetti, L.; Cereda, M.; Monteverde, L.; Desai, N.; Ciccarelli, F.D. Synthetic Lethal Interaction between the Tumour Suppressor STAG2 and Its Paralog STAG1. Oncotarget 2017, 8, 37619. https://doi.org/10.18632/oncotarget.16838.
- Zhu, Q.; Chen, X.; Lin, Z. Discovery of KPT-6566 as STAG1/2 Inhibitor Sensitizing PARP and NHEJ Inhibitors to Suppress Tumor Cells Growth in Vitro. DNA Repair 2024, 144, 103784. https://doi.org/10.1016/j.dnarep.2024.103784.
- Memar, M.Y.; Yekani, M.; Celenza, G.; Poortahmasebi, V.; Naghili, B.; Bellio, P.; Baghi, H.B. The Central Role of the SOS DNA Repair System in Antibiotics Resistance: A New Target for a New Infectious Treatment Strategy. Life Sci. 2020, 262, 118562. https://doi.org/10.1016/j.lfs.2020.118562.
- Kowalczykowski, S.C.; Dixon, D.A.; Eggleston, A.K.; Lauder, S.D.; Rehrauer, W.M. Biochemistry of Homologous Recombination in Escherichia Coli. Microbiol. Rev. 1994, 58, 401–465. https://doi.org/10.1128/mr.58.3.401-465.1994.
- Zhang, X.; Zhou, Z.; Dai, L.; Chao, Y.; Liu, Z.; Huang, M.; Qu, Q.; Lin, Z. Cryo-EM Structure of the RuvAB-Holliday Junction Intermediate Complex from Pseudomonas Aeruginosa. Front. Plant Sci. 2023, 14. https://doi.org/10.3389/fpls.2023.1139106.
- Dai, L.; Lu, L.; Zhang, X.; Wu, J.; Li, J.; Lin, Z. Identification of Small-Molecule Inhibitors of the DNA Repair Proteins RuvAB from Pseudomonas Aeruginosa. Bioorg. Med. Chem. 2022, 73, 117022. https://doi.org/10.1016/j.bmc.2022.117022.
- Bonde, N.J.; Wood, E.A.; Myers, K.S.; Place, M.; Keck, J.L.; Cox, M.M. Identification of recG Genetic Interactions in Escherichia Coli by Transposon Sequencing. J. Bacteriol. 2023, 205, e00184-23. https://doi.org/10.1128/jb.00184-23.
- Lloyd, R.G.; Sharples, G.J. Processing of Recombination Intermediates by the RecG and RuvAB Proteins of Escherichia Coli. Nucleic Acids Res. 1993, 21, 1719–1725.
- Lloyd, R.G.; Buckman, C. Genetic Analysis of the recG Locus of Escherichia Coli K-12 and of Its Role in Recombination and DNA Repair. J. Bacteriol. 1991, 173, 1004–1011. https://doi.org/10.1128/jb.173.3.1004-1011.1991.
- Donaldson, J.R.; Courcelle, C.T.; Courcelle, J. RuvAB and RecG Are Not Essential for the Recovery of DNA Synthesis Following UV-Induced DNA Damage in Escherichia Coli. Genetics 2004, 166, 1631–1640. https://doi.org/10.1534/genetics.166.4.1631.
- Rudolph, C.J.; Upton, A.L.; Briggs, G.S.; Lloyd, R.G. Is RecG a General Guardian of the Bacterial Genome? DNA Repair 2010, 9, 210–223. https://doi.org/10.1016/j.dnarep.2009.12.014.
- Li, L.; Guo, B.; Dai, L.; Liu, C.; Lin, Z. Ebselen and TPI-1, as RecG Helicase Inhibitors, Potently Enhance the Susceptibility of Pseudomonas Aeruginosa to DNA Damage Agents. Biochem. Pharmacol. 2024, 222, 116051. https://doi.org/10.1016/j.bcp.2024.116051.
- Shalem, O.; Sanjana, N.E.; Zhang, F. High-Throughput Functional Genomics Using CRISPR-Cas9. Nat. Rev. Genet. 2015, 16, 299. https://doi.org/10.1038/nrg3899.
- Clements, K.E.; Schleicher, E.M.; Thakar, T.; Hale, A.; Dhoonmoon, A.; Tolman, N.J.; Sharma, A.; Liang, X.; Kawasawa, Y.I.; Nicolae, C.M.; et al. Identification of Regulators of Poly-ADP-Ribose Polymerase Inhibitor Response through Complementary CRISPR Knockout and Activation Screens. Nat. Commun. 2020, 11, 6118. https://doi.org/10.1038/s41467-020-19961-w.
- Pettitt, S.J.; Krastev, D.B.; Brandsma, I.; Dréan, A.; Song, F.; Aleksandrov, R.; Harrell, M.I.; Menon, M.; Brough, R.; Campbell, J.; et al. Genome-Wide and High-Density CRISPR-Cas9 Screens Identify Point Mutations in PARP1 Causing PARP Inhibitor Resistance. Nat. Commun. 2018, 9, 1849. https://doi.org/10.1038/s41467-018-03917-2.
- Choudhury, S.R.; Cui, Y.; Lubecka, K.; Stefanska, B.; Irudayaraj, J. CRISPR-dCas9 Mediated TET1 Targeting for Selective DNA Demethylation at BRCA1 Promoter. Oncotarget 2016, 7, 46545. https://doi.org/10.18632/oncotarget.10234.
- Andronikou, C.; Burdova, K.; Dibitetto, D.; Lieftink, C.; Malzer, E.; Kuiken, H.J.; Gogola, E.; Chaudhuri, A.R.; Beijersbergen, R.L.; Hanzlikova, H.; et al. PARG-Deficient Tumor Cells Have an Increased Dependence on EXO1/FEN1-Mediated DNA Repair. EMBO J. 2024, 43, 1015. https://doi.org/10.1038/s44318-024-00043-2.
- Burslem, G.M.; Crews, C.M. Proteolysis Targeting Chimeras as Therapeutics and Tools for Biological Discovery. Cell 2020, 181, 102. https://doi.org/10.1016/j.cell.2019.11.031.
- Tejwani, V.; Carroll, T.; Macartney, T.; Bandau, S.; Alabert, C.; Saredi, G.; Toth, R.; Rouse, J. PROTAC-Mediated Conditional Degradation of the WRN Helicase as a Potential Strategy for Selective Killing of Cancer Cells with Microsatellite Instability. Sci. Rep. 2024, 14, 20824. https://doi.org/10.1038/s41598-024-71160-5.
- Alfayomy, A.M.; Ashry, R.; Kansy, A.G.; Sarnow, A.-C.; Erdmann, F.; Schmidt, M.; Krämer, O.H.; Sippl, W. Design, Synthesis, and Biological Characterization of Proteolysis Targeting Chimera (PROTACs) for the Ataxia Telangiectasia and RAD3-Related (ATR) Kinase. Eur. J. Med. Chem. 2024, 267, 116167. https://doi.org/10.1016/j.ejmech.2024.116167.
- Zheng, M.; Huo, J.; Gu, X.; Wang, Y.; Wu, C.; Zhang, Q.; Wang, W.; Liu, Y.; Liu, Y.; Zhou, X.; et al. Rational Design and Synthesis of Novel Dual PROTACs for Simultaneous Degradation of EGFR and PARP. J. Med. Chem. 2021, 64, 7839–7852. https://doi.org/10.1021/acs.jmedchem.1c00649.
- Huang, D.; Yang, M.; Wen, X.; Xia, S.; Yuan, B. Ai-Driven Drug Discovery: Accelerating the Development of Novel Therapeutics in Biopharmaceuticals. J. Knowl. Learn. Sci. Technol. 2024, 3, 206–224. https://doi.org/10.60087/jklst.vol3.n3.p.206-224.
- Zhou, S.; Chai, D.; Wang, X.; Neeli, P.; Yu, X.; Davtyan, A.; Young, K.; Li, Y. AI-Powered Discovery of a Novel P53-Y220C Reactivator. Front. Oncol. 2023, 13, 1229696. https://doi.org/10.3389/fonc.2023.1229696.
- Subramanian, N.; Maignan, N.; Tieo, G.; Papazian, D.; Dawe, J.; Georges, M.; de Souza, C.H.Q.; Ravinder, R.; Martin, S.; Jeitany, M. 103P Using AI to Break New Ground in Oncological Drug Discovery: Rapid Identification of Novel Targets and Polypharmacological Compounds for Effective Liposarcoma Treatment. ESMO Open 2024, 9. https://doi.org/10.1016/j.esmoop.2024.102492.
- Rigoni, D.; Yaddehige, S.; Bianchi, N.; Sperduti, A.; Moro, S.; Taccioli, C. TumFlow: An AI Model for Predicting New Anticancer Molecules. Int. J. Mol. Sci. 2024, 25, 6186. https://doi.org/10.3390/ijms25116186.
- Edwards, S.L.; Brough, R.; Lord, C.J.; Natrajan, R.; Vatcheva, R.; Levine, D.A.; Boyd, J.; Reis-Filho, J.S.; Ashworth, A. Resistance to Therapy Caused by Intragenic Deletion in BRCA2. Nature 2008, 451, 1111–1115. https://doi.org/10.1038/nature06548.
- Leslie, E.M.; Deeley, R.G.; Cole, S.P.C. Multidrug Resistance Proteins: Role of P-Glycoprotein, MRP1, MRP2, and BCRP (ABCG2) in Tissue Defense. Toxicol. Appl. Pharmacol. 2005, 204, 216–237. https://doi.org/10.1016/j.taap.2004.10.012.
- McGranahan, N.; Swanton, C. Clonal Heterogeneity and Tumor Evolution: Past, Present, and the Future. Cell 2017, 168, 613–628. https://doi.org/10.1016/j.cell.2017.01.018.
- Scott, L.J. Niraparib: First Global Approval. Drugs 2017, 77, 1029–1034. https://doi.org/10.1007/s40265-017-0752-y.
- Syed, Y.Y. Rucaparib: First Global Approval. Drugs 2017, 77, 585–592. https://doi.org/10.1007/s40265-017-0716-2.