Downloads
Download


This work is licensed under a Creative Commons Attribution 4.0 International License.
Review
A Novel Concept: Utilizing Curtailed Wind and Solar Power for Straw Crushing to Achieve Biomass Energy Storage
Xiying Zhou 1, Bing Hu 1, Huan Zhang 1, Yuguang Zhou 2, Hongqiong Zhang 3, Quanguo Zhang 4, and Zhiping Zhang 1,*
1 Henan International Joint Laboratory of Biomass Energy and Nanomaterials, Henan Agricultural University, No. 63 Wenhua Road, Zhengzhou 450002, China
2 College of Engineering, China Agricultural University, No. 17 Qinghua Donglu, Beijing 100083, China
3 College of Engineering, Northeast Agricultural University, No. 600 Changjiang Street, Xiangfang District, Harbin 150030, China
4 Modern Agricultural Engineering Research Institute, Huanghe S&T University, No.666 Zijingshan South Road, Zhengzhou 450044, China
* Correspondence: zhangzhiping715@163.com
Received: 30 October 2024; Revised: 20 March 2025; Accepted: 21 March 2025; Published: 2 April 2025
Abstract: With various countries setting strategic goals for peaking carbon emissions and achieving carbon neutrality, the global demand for clean energy is showing an increasing trend. On the one hand, wind and solar energy, as the two main pillars of renewable energy, are widely promoted due to their clean and low-carbon environmental benefits. However, the intermittency and instability of these two types of energy have become the primary causes of challenges in new energy consumption and grid integration. On the other hand, a large amount of agricultural waste is produced globally each year, and biomass energy has huge potential. However, in our country, agricultural waste cannot be effectively utilized, one of the important reasons is that the transportation of raw materials is difficult, and some power plants opt to pulverize straw before transporting it, but the straw crushing consumes a lot of energy. This article proposes an innovative model: The straw-crushing plant is combined with the wind power station, and the straw is crushed by abandoning wind and light. This collaborative energy storage mode will effectively alleviate the dual problems of new energy consumption and agricultural waste management. This article, through the analysis of relevant data research indicates that provinces represented by Henan, Hebei, and Shandong not only boast abundant straw resources but also lead in total installed wind and solar power capacity. Rough estimates reveal that Henan Province wastes 1.2 billion kWh of wind and solar power annually, while Hebei Province discards 4 billion kWh yearly. This curtailed wind-solar-straw energy storage system can increase renewable energy utilization efficiency by 3–4%. Compared to traditional grid-based crushing methods, it reduces energy costs for straw pretreatment by 30–40% and achieves a 15–20% reduction in carbon emissions over its full lifecycle. This system offers an innovative approach to integrating renewable energy integration with agricultural circular economy development. It holds a certain guiding significance for the field of new energy consumption and storage.
Keywords:
biomass energy storage straw crushing curtailed wind and solar power biomass materials1. Introduction
Energy is the foundation for driving social progress. With the depletion of traditional energy sources such as fossil fuels and their adverse environmental impact [1], countries worldwide are actively developing renewable energy sources, which include the exploitation and utilization of wind, solar, hydropower, and biomass resources. Due to the addition of various forms of power supply such as photovoltaic and wind power, China’s electricity continues to maintain a high-capacity level, but also thanks to the diversification and cleanliness of electricity forms, China’s new energy automobile industry has a strong momentum of development [2]. In addition to the use of wind and solar energy, China’s biomass energy development potential is also very large [3]. Biomass resources are diverse and abundant, with a large amount of agricultural waste biomass generated globally each year, including straw, dead branches, fallen leaves, etc. [4]. The transformation and utilization of biomass resources have become a popular research topic today [5–7]. Many scholars are dedicated to the transformation research of agricultural waste, and the available agricultural waste biomass is used to produce biogas, hydrogen, fertilizer, and new high-value-added materials [8]. Chuen-Shii Chou and colleagues used crushed rice straws and rice husks as raw materials to produce solid fuel briquettes [9]. Meng et al. explored the potential of producing high value-added products by co-pyrolysis of mushroom waste mixed with pine sawdust and wheat straw [10]. Zhang Zhiping investigated ultra-fine grinding of straw and studied the feasibility of hydrogen production using cellulase and photosynthetic hydrogen-producing bacteria [11]. Yang Jiancheng and others utilized the CIP method to treat corn straw, effectively breaking down cellulose and significantly promoting methane production [12]. In China, with its enormous straw output, broad prospects for utilization, and significant economic benefits, especially in the field of biomass energy conversion, straw is transformed into high value-added products through a series of treatments [13,14]. Straw and other agricultural waste contain a large amount of cellulose and hemicellulose, which are globally recognized as difficult to decompose [15,16]. The presence of these substrates significantly increases the difficulty of biomass development and utilization. In the development and utilization of biomass resources, especially the utilization of lignocellulosic biomass, whether it is energy conversion to produce biomethane [17] and biohydrogen, or material conversion to produce carbon fiber reinforced materials that can be used in the automotive industry, throughout the entire conversion process, the crushing process plays a crucial role [18]. Crushing not only improves the bulk density of straw [19], reducing transportation costs, but also fragments the straw into fine particles. The physical and chemical properties of lignocellulosic biomass are changed, such as the specific surface area increasing, etc., so that it shows stronger biological accessibility and material properties. Therefore, optimizing the straw crushing process and reducing related energy consumption is of significant importance for enhancing the economic viability and environmental sustainability of biomass energy conversion. On the synergistic integration of wind/solar power with complementary energy storage technologies, Xuecen Zhang et al. investigated the co-location strategy of integrating curtailed wind power with compressed air energy storage (CAES) to mitigate wind curtailment in the UK power system. Their study demonstrated this approach enhances wind power dispatchability through energy time-shifting, but highlighted CAES’s inherent limitations in providing fast-ramping capabilities to address sub-hourly wind power fluctuations [20]. Jingze Yang et al. optimized the design parameters for utilizing curtailed wind and solar power in hydrogen production via water electrolysis under varying operational conditions. Their analysis demonstrated a levelized hydrogen production cost of 7.94 USD/kg at a hydrogen supply loss probability of 0.1. However, the study failed to address the local water resource availability and lacked a comprehensive economic analysis of water consumption for electrolysis processes—a critical oversight given that water requirements typically range from 9 to 12 L per kg of hydrogen produced in commercial PEM electrolyzers [21].
2. Application of Biomass Resources in Automotive Industry
2.1. The Application of Bio-Oil in Automotive Industry
Biofuels are derived from renewable organic matter such as corn, sugar cane, and algae [22]. Compared to conventional fossil fuels, their combustion releases fewer greenhouse gases and contributes more to reducing overall carbon emissions [23]. In recent years, with the acceleration of the dual-carbon strategy, the application of biofuels in the automotive industry has become a hot topic. Adding biofuels to the automotive industry system can reduce dependence on fossil fuels and improve the security and stability of the energy supply. Federico Millo et al. studied the impact of mixed diesel and ordinary diesel added with biofuels on the performance of automobile engines and found that under specific conditions, diesel added with biofuels can reduce the emission of carbon monoxide and hydrocarbon mixture [24]. Although the performance and efficiency of the engine using biomass fuel will be reduced, the characteristics of low sulfur oxide emissions and low particulate pollutants (PM) emissions are still worthy of further study [25]. Biomass fuel made from algae is the second-generation fuel, and compared with other biomass, algae show great advantages in cost and scale application [26]. However, in some countries, biomass fuel still has some problems in raw materials. Luke O. Ajuka et al. took Nigeria as an example to demonstrate the challenges of using cassava waste as biomass fuel in the country, including insufficient cassava production and large planting areas [27]. On the other hand, many studies have pointed to the modification of biofuels for a wider range of application scenarios. Borman studied the characteristics of rapeseed oil after hydrotreating, and this modified biomass fuel is more suitable for traditional cars and trucks [28]. In addition, some researchers mixed biofuels derived from plants such as Jatropha curcas with commercial diesel and compared the exhaust temperature, smoke density, carbon monoxide (CO), hydrocarbon (HC), carbon dioxide (CO2), and nitrogen oxide (NOx) emissions of raw diesel and different proportions of biodiesel under different loads. The results show that when jatropha curcas oil is mixed with commercial diesel, the nitrogen oxide emission of pure biodiesel is significantly reduced, and it has lower specific fuel consumption and higher braking thermal efficiency [29]. Overall, biofuels have great potential in automotive applications and may play an important role in the near future, and the application of biofuels in the automotive industry is a viable way to achieve the Sustainable Development Goals. Of course, there are still problems of low combustion efficiency, and inconvenient preparation, storage, and transportation of biomass fuel. While challenges remain, continued technological advances and policy support are expected to ease these barriers. As the industry continues to evolve, the role of biofuels is likely to expand, contributing to a greener and more resilient automotive future.
2.2. Application of Bio-Based Materials in the Automotive Industry
In recent years, with the increasing demand for sustainable and environmentally friendly materials in various industries, driven by reducing carbon footprint and reducing dependence on fossil fuels, the application of bio-based plastics in the automotive industry has also received increasing attention. Bio-based plastics, with their low carbon footprint and low pollution, can provide solutions to the environmental challenges posed by conventional plastics [30]. For example, bio-based food plastics, bio-based industrial materials, etc., the development of bio-based plastic materials is crucial for the sustainable development of various industries [31]. Manggar Arum Aristri et al. studied tannin obtained from biomass to produce PU, which can be used to improve the mechanical and thermal properties of materials in automotive and other industries [32]. In view of the application limitations of biobased materials, Tizazu Mekonnen et al. introduced the performance improvement of common plasticizers on biobased materials [33]. Elisa Zini et al. gave a comprehensive overview of green composites, focusing on the advantages of natural fiber-based polymer composites and bio-based polymers in environmental protection. The development of thermoplastic and thermosetting biobased polymers has provided new options for sustainable materials in the automotive manufacturing industry. The application potential of lignin as a reinforcing agent for plastic composites was also discussed [34]. Dias et al. prepared samples mixed with lignin powder and polypropylene. Through physical, mechanical, and thermal properties tests, the results show that the lignin powder-added polypropylene composites exhibit excellent properties and are suitable for various industrial applications, especially in the field of engineering plastics replacement where critical mechanical properties are required, demonstrating the mechanical properties potential of bio-based plastics in automotive applications [35]. Taiqu Liu et al. studied coconut peel as the core material of the composite sandwich structure and compared the energy absorption behavior of glass fiber-reinforced plastic plate and carbon fiber-reinforced composite plate. The results show that the energy absorption per unit weight of sandwich structures with glass fiber-reinforced plastic plates is higher than that of carbon fiber-reinforced composite plates, which proves the superiority of these new biocomposites in terms of energy absorption and mechanical properties [36]. Overall, the shift from traditional plastics to bio-based plastics offers new opportunities for global economic growth and sustainable development, especially in key areas such as automotive engineering. This shift will not only help reduce dependence on fossil resources but also promote the development of environmentally friendly products and push the industry in a greener, low-carbon direction [37]. By using bio-based materials instead of traditional materials, the automotive industry can contribute to a greener environment and low-carbon economy, bringing brighter prospects for automotive development.
3. Research Status of Straw Crushing Technology
Currently, the primary methods of straw crushing technology are mechanical and physical [38,39], such as hammer milling: where hammers shatter the straw through impact force during high-speed rotation. The shape and quantity of the hammers can be selected and adjusted according to the type and moisture content of the straw to achieve the desired crushing effect [40,41]. This method typically requires the hammers to rotate at high speeds and with a certain amount of force, and it is usually effective for materials with larger particles. Ball milling: Similar in principle to hammer milling, grinding balls collide and compress the material to induce strain, thereby achieving material fragmentation [42]. This method usually yields a sample that is ground thoroughly and uniformly. Blade crushing: This involves cutting the material through the high-speed rotation of blades. For instance, Lang Wen and others used a crusher to process straw in their study on incorporating straw into concrete experiments, obtaining suitable samples [43]. This method is relatively common but is only applicable to materials after drying. Most of these straw-crushing methods share a common problem: the more uniform and finer the straw sample, the higher the energy consumption required. Taking the laboratory Baixin LG-500A crusher as an example, with a power of 1.4 kW, a capacity of 500 g, and a speed of 25,000 rpm, crushing straw to 200 mesh consumes about 1 kWh of electricity for processing around 10 kg of straw. 1 kWh equals 3.6 MJ of energy, while the complete combustion of 10 kg of straw can release 140–150 MJ of energy, under normal circumstances, it is possible to produce 0.33 cubic meters of hydrogen. The world generates over 2 billion tons of straw annually. Calculating this figure, crushing all this straw would require 2 trillion kilowatt-hours of electricity, which is incredible. If the sample is required to be too fine, the energy consumed by electric power and other processes may exceed the biomass energy that can be converted, which is economically irrational. However, the finer the straw, the greater its bulk density, which results in a faster reaction rate in hydrogen production reactions and yields better value-added products. This phenomenon is called the “trade-off between crushing degree and reaction rate” [44].
These two factors balance the development and utilization of biomass energy, becoming a bottleneck in the current development of biomass energy. Future research needs to focus on developing new low-energy crushing equipment and finding sources of low-cost electricity to achieve energy-efficient and high-efficiency biomass crushing processes.
4. The Application of Wind and Photovoltaic Power in Biomass Crushing
Wind and photovoltaic power, as important components of renewable energy, have extremely abundant reserves. The common characteristics of these two types of energy are that they are clean, renewable, and have extremely low carbon emissions, making them very suitable for replacing traditional fossil fuel energies. In recent years, the total installed capacity of wind and solar power across the country has grown rapidly. The widespread promotion of wind and solar power generation has effectively eased the global warming problem caused by the combustion of fossil fuels, holding significant importance for dual-carbon strategic goals. However, wind and solar power generation are greatly affected by regional environmental factors, resulting in fluctuations in power generation, difficulties in grid connection, and issues with consumption, also known as the problems of wind and solar power curtailment [45,46]. Wind and solar power curtailment refers to the phenomenon where electrical energy generated from wind and photovoltaic power cannot be consumed due to grid load limitations or insufficient transmission capacity. This phenomenon is particularly common in regions where wind and photovoltaic power are rapidly developing [47]. The existence of wind and solar power curtailment not only reduces the utilization efficiency of renewable energy but also diminishes the economic benefits of investment. According to the latest data released by the National Renewable Energy Consumption Monitoring and Early Warning Center, in the first half of 2024, China’s wind power utilization rate reached 96.1%, and the photovoltaic power generation utilization rate was 97.0%. Despite significant progress in new energy consumption since the introduction of relevant national policies in 2018, new challenges continue to emerge amidst constantly changing market and technological environments [48].
To address these challenges, the development strategy of combining wind and solar power generation with energy storage technology is considered a long-term effective solution to the energy consumption problem [49–51]. However, due to factors such as technological levels, the construction of traditional energy storage facilities is enormously costly. The average storage cost per kWh in 2020 was 0.5 yuan, approximately equal to the electricity price per kWh. In some regions, the cost of energy storage investment and construction per kilowatt-hour even exceeded the price per kilowatt-hour. This implies that the payback period for energy storage is relatively long. In China, pumped hydro storage accounts for 90% of the energy storage market [52,53], suitable for hydropower generation, while the share of storage for photovoltaic and wind power is only about 10%. The primary reason for this situation is the high cost of building electrochemical energy storage facilities and the long payback period for investments, which constrains the development of the energy storage industry. In response to this issue, this article proposes a new model: constructing straw-crushing plants near wind and photovoltaic power plants, utilizing unconsumed energy to crush straw. On one hand, this solves the renewable energy consumption issue; on the other hand, it addresses the high energy consumption problem of straw crushing. The idea of using curtailed wind and solar power for biomass crushing is based on recognizing the potential value of these underutilized electrical energies. This not only improves the overall utilization rate of wind and photovoltaic power but also provides a low-cost, low-carbon energy solution for biomass crushing.
5. Feasibility Analysis of Biomass Energy Storage Using Wind and Solar Power Generation
In order to actively respond to the dual-carbon strategy, many provinces across the country have accelerated the construction of new energy, and the total installed capacity of wind power and solar power generation has continued to reach a new level. This paper collects data on wind and solar installations and straw production in key provinces, conducting a feasibility analysis of biomass energy storage using curtailed wind and solar power from the perspectives of geographical feasibility and economic viability.
As shown in Figure 1, in 2021, provinces with a total installed wind and solar capacity of over 20 million kilowatts included Hebei, Shandong, and Inner Mongolia, which each exceeded 50 million kilowatts. Influenced by terrain, regions east of the Taihang Mountains and along the southeast coast, including Hebei, Henan, Guangdong, Zhejiang, and other provinces and cities, are rich in wind energy resources. Affected by weather patterns, photovoltaic power generation is concentrated in Xinjiang, Qinghai, and other regions. As shown in Figure 2, the total volume of straw resources across major regions of the country is primarily distributed in North China, Northeast China, and the middle and lower reaches of the Yangtze River [54]. These areas coincidentally are the main grain-producing regions of the country, with Heilongjiang, Hebei, Shandong, Henan, and others ranking at the top in terms of straw volume nationwide. These regions boast not only abundant wind and solar resources but also plentiful straw resources. Therefore, in regions such as Henan and Hebei, it is feasible to utilize curtailed wind and solar power for straw crushing and biomass energy storage.
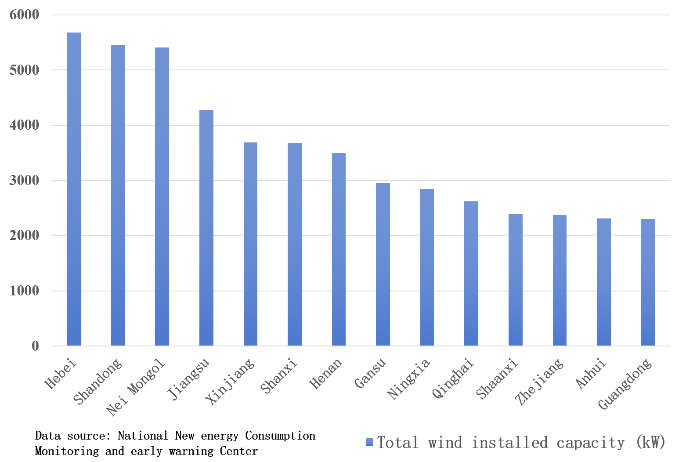
Figure 1. Provinces with straw production exceeding 20 million tons in 2021.
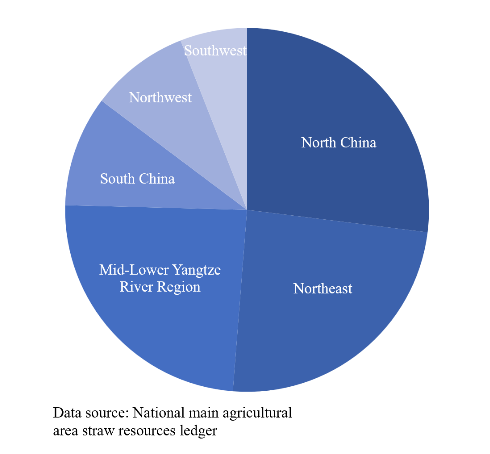
Figure 2. Straw production by region.
In 2023, Henan Province had a wind power utilization rate of 96.8% and a photovoltaic power utilization rate of 97.7%, with the total annual wind and solar power generation reaching 40 billion kWh. After calculation, it was determined that in 2023, Henan Province wasted over 1.2 billion kWh of electricity due to curtailed wind and solar power. This amount of electricity could have been used to crush at least 12 million tons of straw. According to statistics, in 2023, the total production of various crop straws in Henan Province reached more than 79 million tons. If calculated at 0.5 yuan per kWh, collecting this curtailed wind and solar power could save at least 600 million yuan in electricity expenditures for straw crushing, and additionally generate 600 million yuan in extra revenue for power plants. In 2023, Hebei Province had a wind and solar power utilization rate of 94.3%, and a photovoltaic power utilization rate of 97.5%, with the total annual wind and solar power generation reaching 83.7 billion kWh. However, the abandonment of wind and solar power resulted in a waste of 4 billion kWh of electricity. This curtailed energy could have saved at least 2 billion yuan in expenses and resolved the issue of curtailed wind and solar power. Utilizing curtailed wind and solar power for straw crushing and biomass energy storage is very feasible economically.
6. Future Perspectives
The implementation of this comprehensive system requires advancements in three key areas:
Technological Innovation: Develop AI-based prediction systems for wind curtailment forecasting, automatically monitor grid-side loads, and regulate power supply for crusher operations to maximize the efficiency of wind and solar power generation.
Policy Framework: Encourage enterprises to develop biomass energy storage projects utilizing curtailed wind and solar energy, and promote demonstration projects. Support the integration of such projects into the national carbon trading market.
International Standardization: Establish ISO certification standards for bioenergy storage efficiency metrics (e.g., kWh/ton-CE, carbon sequestration index).
7. Conclusions
Through extensive research and sound argumentation, the idea of “Biomass Energy Storage”—utilizing discarded wind and solar power to crush straw, reducing its bulk density, and indirectly storing electrical energy within the biomass is raised. This model effectively lowers transportation costs, enhances subsequent reaction rates, and improves the utilization rate of wind and solar power generation. This paper discusses the feasibility of using discarded wind and solar power for biomass energy storage from both geographic and economic perspectives. The results indicate that this model not only significantly improves the utilization rate of wind and solar energy but also reduces energy consumption during biomass processing, decreases the carbon footprint of the entire agricultural process, and promotes rapid development in biomass energy storage and resource utilization. It has a positive significance for achieving dual carbon strategic goals.
Inspired by pumped-storage hydroelectricity, our team noticed the high energy consumption issue in straw crushing during daily experiments. Finding a breakthrough in discarded wind and solar power, we surveyed straw production in major provinces and cities across the country and collected data on the utilization rate of wind and solar power generation in various regions. We argued for the rationality of discarded wind and solar power storage from two main aspects, providing new insights for promoting sustainable energy development. In the future, with technological advancements and policy support, this strategy is expected to be more widely applied and promoted, making a significant contribution to achieving dual carbon strategic goals.
Author Contributions: Conceptualization, Z.Z. and Y.Z.; methodology, B.H.; validation, H.Z. and H.Z.; formal analysis, Q.Z.; investigation, X.Z.; writing—original draft preparation, X.Z.; project administration, Z.Z. All authors have read and agreed to the published version of the manuscript.
Funding: This work was financially supported by the Natural Science Project of Henan Province (Grant No. 232300421063), Key Research and Development Program Project of Henan Province (251111110100), Youth Top Talent of Central Plains, Henan Province and the Science and Technology Project of the First Division of Alar (2022TF02).
Institutional Review Board Statement: Not applicable.
Informed Consent Statement: Not applicable.
Data Availability Statement: Not applicable.
Conflicts of Interest: The authors declare no conflict of interest.
References
- Wang, Z.; Zeng, S.; Khan, Z. Impact of sustainable energy, fossil fuels and green finance on ecosystem: Evidence from China. Heliyon 2024, 10, 1005–1019.
- Tian, J.; Wang, P.; Zhu, D. Overview of Chinese new energy vehicle industry and policy development. Green Energy Resour. 2024, 2, 100075.
- Zhang, J.; Wei, J.; Guo, C.L.; Tang, Q.; Guo, H. The spatial distribution characteristics of the biomass residual potential in China. J. Environ. Manag. 2023, 338, 117777.
- Saleem, M. Possibility of utilizing agriculture biomass as a renewable and sustainable future energy source. Heliyon 2022, 8, e08905.
- Gu, F.; Gao, X.; Wu, F.; Hu, Z.; Chen, Y.; Zhang, C. Improving Uniform Scattering Device for Straw-smashing, Back-throwing, No-tillage Planter Under Complete Straw Mulching Condition. Int. J. Agric. Biol. Eng. 2018, 11, 49–57.
- Shi, Y.; Rex, S.X.; Wang, X.; Hu, Z.; Newman, D.; Ding, W. Numerical Simulation and Field Tests of Minimum-tillage Planter with Straw Smashing and Strip Laying Based on EDEM Software. Comput. Electron. Agric. 2019, 166, 105021.
- Xu, Y.; Zhang, X.; Wu, S.; Chen, C.; Wang, J.; Yuan, S.; Chen, B.; Li, P.; Xu, R. Numerical Simulation of Particle Motion at Cucumber Straw Grinding Process Based on EDEM. Int. J. Agric. Biol. Eng. 2020, 13, 227–235.
- Wang, J.; Hou, D.; Liu, Z.; Tao, J.; Yan, B.; Liu, Z.; Yang, T.; Su, H.; Tahir, M.H.; Chen, G. Emergy analysis of agricultural waste biomass for energy-oriented utilization in China: Current situation and perspectives. Sci. Total Environ. 2022, 894, 157798.
- Chou, C.-S.; Lin, S.-H.; Lu, W.-C. Preparation and characterization of solid biomass fuel made from rice straw and rice bran. Fuel Process. Technol. 2009, 90, 980–987.
- Meng, H.; Yang, H.; Wu, Z.; Li, D.; Wang, Z.; Wang, D.; Wang, H.; Li, H.; Li, J. Co-Pyrolysis of Mushroom Residue Blended with Pine Sawdust/Wheat Straw for Sustainable Utilization of Biomass Wastes: Thermal Characteristics, Kinetic/Thermodynamic Analysis, and Structure Evolution of Co-Pyrolytic Char. Sustainability 2024, 16, 6677.
- Zhang, Z.P. Study on the Feasibility of Ultrafine Pretreatment Technology of Straw Biomass and Its Hydrogen Production. Master’s Thesis, Henan Agricultural University, Zhengzhou, China, 2012. (In Chinese)
- Yang, J.; Chen, R.; Zhang, Q.; Zhang, L.; Li, Q.; Zhang, Z.; Wang, Y.; Qu, B. Green and chemical-free pretreatment of corn straw using cold isostatic pressure for methane production. Sci. Total Environ. 2023, 897, 165442.
- Tiwari, M.; Vinu, R. Microwave-assisted co-pyrolysis of rice straw pellets and plastic packaging wastes for value-added products and energy recovery. Process Saf. Environ. Prot. 2024, 190, 606–621.
- Singh, Y.; Sharma, S.; Kumar, U.; Sihag, P.; Balyan, P.; Singh, K.P.; Dhankher, O.P. Strategies for economic utilization of rice straw residues into value-added by-products and prevention of environmental pollution. Sci. Total Environ. 2024, 906, 167714.
- Campbell, M.M.; Sederoff, R.R. Variation in Lignin Content and Composition Mechanisms of Control and Implications for The Genetic Improvement of Plants. Plant Physiol. 1996, 110, 3–13.
- Zhao, X.; Liu, P. Focus on bioenergy industry development and energy security in China. Renew. Sustain. Energy Rev. 2014, 32, 302–312.
- Stanley, J.T.; Thanarasu, A.; Kumar, P.S.; Periyasamy, K.; Raghunandhakumar, S.; Periyaraman, P.; Devaraj, K.; Dhanasekaran, A.; Subramanian, S. Potential pre-treatment of lignocellulosic biomass for the enhancement of biomethane production through anaerobic digestion—A review. Fuel 2022, 318, 123593.
- Li, Y.; Yan, F.; Li, T.; Zhou, Y.; Jiang, H.; Qian, M.; Xu, Q. High-solid anaerobic digestion of corn straw for methane production and pretreatment of bio-briquette. Bioresour. Technol. 2018, 250, 741–749.
- Rezaei, H.; Tajilrou, M.; Lee, J.S.; Singaraveloo, K.; Lau, A.; Sokhansanj, S. Evolution of biomass particles during pelletization process. Particuology 2024, 86, 182–187.
- Zhang, X.; Jenne, S.P.; Ding, Y.; Spencer, J.; He, W.; Wang, J. A wind power curtailment mitigation strategy via co-location and co-operation of compressed air energy storage with wind power generation. Electr. Power Syst. Res. 2025, 241, 111318.
- Yang, J.; Chi, H.; Cheng, M.; Dong, M.; Li, S.; Yao, H. Performance analysis of hydrogen supply using curtailed power from a solar-wind-storage power system. Renew. Energy 2023, 212, 1005–1019.
- Rudra, S.; Akhtar, T. Algal Biomass Conversion: Hydrothermal Liquefaction for Advanced Bio-Fuel Production. In Encyclopedia of Renewable Energy, Sustainability and the Environment, 1st ed.; Rahimpour, M.R., Ed.; Elsevier: Amsterdam, the Netherlands, 2024; pp. 745–762.
- Lewis, N.G.; Yamamoto, E. Lignin: Occurrence, Biogenesis and Biodegradation. Annu. Rev. Plant Physiol. Plant Mol. Biol. 1990, 41, 455–496.
- Millo, F.; Bensaid, S.; Fino, D.; Marcano, S.J.C.; Vlachos, T.; Debnath, B.K. Influence on The Performance and Emissions of An Automotive Euro 5 Diesel Engine Fueled with F30 from Farnesane. Fuel 2014, 138, 134–142.
- Sentanuhady, J.; Atmaja, G.P.S.G.; Muflikhun, M.A. Challenges of Biofuel Applications in Industrial and Automotive: A Review. J. Eng. Sci. Technol. Rev. 2021, 14, 119–134.
- Chen, H.; Qiu, T.; Rong, J.; He, C.; Wang, Q. Microalgal Biofuel Revisited: An Informatics-based Analysis of Developments to Date and Future Prospects. Appl. Energy 2015, 155, 585–598.
- Ajuka, L.O. Biofuel Resources Plan: Theoretical Case Assessment of Automotive Industries. Eng. Technol. J. 2021, 6, 985–1001.
- Borman, S. Synthesized Biofuel Meets Vehicle Standards. Chem. Eng. News 2017, 95, 10–11.
- Manivannan, A.; Prabu, R.; Kumar, K.M. Investigation on Influence of Blending Jatropha Biofuel with Diesel to Improve Fuel Quality. Aust. J. Mech. Eng. 2019, 19, 49–56.
- Jeremic, S.; Milovanovic, J.; Mojicevic, M.; Skaro-Bogojevic, S.; Nikodinovic-Runic, J. Understanding Bioplastic Materials—Current State and Trends. J. Serbian Chem. Soc. 2020, 85, 1507–1538.
- Coppola, G.; Gaudio, M.T.; Lopresto, C.G.; Calabro, V.; Curcio, S.; Chakraborty, S. Bioplastic from Renewable Biomass: A Facile Solution for A Greener Environment. Earth Syst. Environ. 2021, 5, 231–251.
- Aristri, M.A.; Lubis, M.A.R.; Iswanto, A.H.; Fatriasari, W.; Sari, R.K.; Antov, P.; Gajtanska, M.; Papadopoulos, A.N.; Pizzi, A. Bio-Based Polyurethane Resins Derived from Tannin: Source, Synthesis, Characterisation, and Application. Forests 2021, 12, 1516.
- Mekonnen, T.; Mussone, P.; Khalil, H.; Bressler, D. Progress in Bio-based Plastics and Plasticizing Modifications. J. Mater. Chem. 2013, 1, 13379–13398.
- Zini, E.; Scandola, M. Green Composites: An Overview. Polym. Compos. 2011, 32, 1905–1915.
- Dias, O.A.T.; Negrão, D.R.; Silva, R.C.; Funari, C.S.; Cesarino, I.; Leao, A.L. Studies of Lignin as Reinforcement for Plastics Composites. Mol. Cryst. Liq. Cryst. 2016, 628, 72–78.
- Liu, T.; Hou, S.; Nguyen, X.; Han, X. Energy Absorption Characteristics of Sandwich Structures with Composite Sheets and Bio Coconut Core. Compos. Part B-Eng. 2017, 114, 328–338.
- Esposti, M.D.; Morselli, D.; Fava, F.; Bertin, L.; Cavani, F.; Viaggi, D.; Fabbri, P. The Role of Biotechnology in The Transition from Plastics to Bioplastics: An Opportunity to Reconnect Global Growth with Sustainability. Febs Open Bio 2020, 11, 967–983.
- Niu, K.; Yang, Q.; Bai, S.; Zhou, L.; Chen, K.; Wang, F.; Xiong, S.; Zhao, B. Simulation Analysis and Experimental Research on Silage Corn Crushing and Throwing Device. Appl. Eng. Agric. 2021, 37, 725–734.
- Fu, J.; Ji, C.; Wang, W.; Liu, H.; Zhang, G.; Gao, Y.; Zhou, Y.; Abdeen, M.A. Design and Test of Smashing and Scattering Device of Double-channel Feeding Ratoon Rice Harvester. Sci. Rep. 2022, 12, 15943.
- Tumuluru, J.S.; Tabil, L.G.; Song, Y.; Iroba, K.L.; Meda, V. Grinding energy and physical properties of chopped and hammer-milled barley, wheat, oat, and canola straws. Biomass Bioenergy 2014, 60, 58–67.
- Jensen, P.D.; Temmerman, M.; Westborg, S. Internal particle size distribution of biofuel pellets. Fuel 2011, 90, 980–986.
- Jiang, Y.; Lu, Y.; Liu, J.; Zhao, Y.; Fan, F. Characterization of bamboo shoot cellulose nanofibers modified by TEMPO oxidation and ball milling method and its application in W/O emulsion. LWT 2024, 205, 116563.
- Wen, L.; Yan, C.; Shi, Y.; Wang, Z.; Liu, G.; Shi, W. Experimental Study on the Compressive Strength of Concrete with Different Wheat Straw Treatment Techniques. J. Renew. Mater. 2023, 11, 3681–3692.
- Dunbar, E.; Graham, R.A.; Holman, G.T.; Anderson, M.U.; Thadhani, N.N. Time-resolved Pressure Measurements in Chemically Reacting Powder Mixtures. High-Press. Sci. Technol. 1994, 309, 1303–1306.
- Coutinho, R.; Hoshima, H.Y.; Vianna, M.T.G.; Marques, M. Sustainable Application of Modified Luffa Cylindrica Biomass for Removal of Trimethoprim in Water by Adsorption with Process Optimization. Environ. Sci. Pollut. Res. Int. 2024, 31, 55280–55300.
- Yu, F.R.; Zhang, P.; Xiao, W.; Choudhury, P. Communication Systems for Grid Integration of Renewable Energy Resources. IEEE Netw. 2011, 25, 22–29.
- Dai, J.; Yang, X.; Wen, L. Development of wind power industry in China: A comprehensive assessment. Renew. Sustain. Energy Rev. 2018, 97, 156–164.
- Estanqueiro, A.; Couto, A. Chapter 1-New electricity markets. The Challenges of Variable Renewable Energy. In Local Electricity Markets; Pinto, T., Vale, Z., Widergren, S., Eds.; Academic Press: Cambridge, MA, USA, 2021; pp. 3–20.
- Al-Shetwi, A.Q.; Abidin, I.Z.; Mahafzah, K.A.; Hannan, M.A. Feasibility of future transition to 100% renewable energy: Recent progress, policies, challenges, and perspectives. J. Clean. Prod. 2024, 478, 143942.
- Han, X.; Lv, F.; Li, J.; Zeng, F. Flexible interactive control method for multi-scenario sharing of hybrid pumped storage-wind-photovoltaic power generation. J. Energy Storage 2024, 100, 113590.
- Yan, J.; Liu, S.; Yan, Y.; Liu, Y.; Han, S.; Zhang, H. How to choose mobile energy storage or fixed energy storage in high proportion renewable energy scenarios: Evidence in China. Appl. Energy 2024, 376, 124274.
- Tian, C.; Tan, Q.; Fang, G.; Wen, X. Hydrogen production to combat power surpluses in hybrid hydro-wind-photovoltaic power systems. Appl. Energy 2024, 371, 123627.
- Zhao, F.; Liu, X.; Zhao, X.; Wang, H. Spatiotemporal distribution pattern and analysis of influencing factors of pumped storage power generation in China. J. Energy Storage 2024, 79, 110078.
- Qian, B.; Shao, C.; Yang, F. Spatial suitability evaluation of the conversion and utilization of crop straw resources in China. Environ. Impact Assess. Rev. 2024, 105, 107438.